1. INTRODUCTION
Owing to the depletion of fossil fuels and the problems associated with their use, such as carbon dioxide emissions and global warming, biomass resources as renewable energy sources are becoming important alternatives to fossil fuels (Bang et al., 2022; Pari et al., 2023). Lignocellulosic biomass has attracted significant attention as a potentially sustainable resource because it can be used to produce a variety of chemicals and fuels (Hwang et al., 2021; Iswanto et al., 2021).
However, it is very difficult to effectively utilize lignocellulosic biomass because it is composed of complex structures, including cellulose, hemicellulose, and lignin (Fatriasari et al., 2020). Therefore, pretreatment is essential for the biochemical conversion processing for bioethanol production and biorefinery from lignocellulosic biomass (Maulana et al., 2021; Zendrato et al., 2021). The main purpose is to analyze, understand, and overcome lignocellulosic biomass recalcitrance, which restricts the degradation of biomass by chemicals and catalysts, as well as the accessibility of enzymes for effective pretreatment (Himmel, 2008; United States Department of Energy, 2006).
Recalcitrance is a natural and unique property of lignocellulosic biomass, including the lignin-carbohydrate complex, cellulose crystallinity, content and acetylation of hemicellulose, content and distribution of lignin, available pore volume distribution, and surface area (United States Department of Energy, 2006). Therefore, to effectively overcome biomass recalcitrance, the conversion characteristics of each component of lignocellulosic biomass during pretreatment should be investigated.
Various pretreatments have been studied for lignocellulosic bioethanol production and biorefineries (da Costa Sousa et al., 2009; Mosier et al., 2005; Sun and Cheng, 2002). Among them, organosolv pretreatment is considered an effective pretreatment method for lignocellulosic biomass containing high lignin content to produce glucose, organosolv lignin, and other useful chemicals, because an organic solvent and an acid catalyst effectively break down internal lignin and hemicellulose bonds (Holtzapple and Humphrey, 1984). In this study, organosolv pretreatment was adopted, and ethanol was used as an organic solvent to enhance softwood impregnation by transferring the catalyst or reagent to lignin. Sulfuric acid was used as a catalyst to promote the enzymatic conversion of biomass into reducing sugar.
The severity factor [Log (Ro)] was developed to account for the effects of temperature and residence time on non-catalyzed aqueous media (Jung et al., 2022; Overend and Chornet, 1987). However, owing to the addition of acid catalysts during the pretreatment processes, the effects of pH were considered. Therefore, the severity depending on temperature and residence time, in addition to pH, was described using the combined severity factor (CSF).
Liriodendron tulipifera, one of the most attractive hardwoods as a renewable and sustainable resource for bioethanol production and biorefineries, was used as the starting material. It is fast-growing, adaptable to a variety of climates and soil conditions, and tolerant to damage caused by harmful insects (Kim et al., 2023). L. tulipifera is a major afforestation tree recommended by the Korea Forest Service, and its application is currently being studied.
In this study, during acid-catalyzed organosolv pretreatment of L. tulipifera, the conversion characteristics of monosaccharides (glucose and xylose) and polysaccharides (cellulose and hemicellulose) were pretreatment of L. tulipifera. Furthermore, its effect on the recalcitrance of lignocellulosic biomass was evaluated.
2. MATERIALS and METHODS
Stems of 20-year-old L. tulipifera (Gangjin, Korea) were pulverized into powder and sieved through a 40-mesh sieve. The materials were stored at 4°C until organosolv pretreatment, and the moisture content was less than 5.0%. Ethanol and sulfuric acid for the organosolv pretreatment were purchased from Samchun Chemical (Pyeongtaek, Korea). Acetic acid and sodium acetate in a sodium acetate buffer were purchased from Samchun Chemical and Duksan Reagent (Seoul, Korea), respectively. Avicel PH-101 as cellulose standard was purchased from Sigma-Aldrich (Yongin, Korea).
Organosolv pretreatment was conducted in a reactor composed of a vessel, an electric band heater, a magnetic drive with a paddle-type impeller, and a control box (HR-8300, Hanwoul Engineering, Gunpo, Korea). The vessel was made of stainless steel (SUS 316) and had a capacity of 1,000 mL. A Teflon gasket was used to maintain the inner pressure. A thermocouple and pressure gauge were located inside the reactor to measure the internal temperature and pressure, respectively, during pretreatment. A Teflon impeller was placed inside the reactor under regular stirring. The control box controlled the temperature of the vessel and heater and the stirring rate of the impeller.
The ratio of the material to solvent was 1:10 (50 g: 500 mL). Ethanol [50% (v/v)] was used as an organic solvent, and sulfuric acid [1.0% (w/w)] was added as a catalyst. The pretreatment was performed with consistent output of the electric band heater until the internal temperature reached the target (120°C, 130°C, 140°C, 150°C, 160°C, 170°C, or 180°C). After the organosolv pretreatment, the vessel was quenched in an ice chamber, and cooled to room temperature.
After being cooled to room temperature, the pretreated materials were filtered through a filter paper (Advantec, Tokyo, Japan) and divided into solid residue and liquid hydrolysate. The solid residue was washed using 1,000 mL of distilled water to remove the constituents that remained in the solid residue. The washed solid residue was lyophilized for structural analyses, and the washed water (hereafter termed washed filtrate) was separately stored to prevent precipitation by mixing with the liquid hydrolysate. A precipitate was formed by mixing the liquid hydrolysate and washed filtrate and was subsequently collected by centrifugation and lyophilization. Finally, the pretreated materials were divided into three fractions: the solid residue, liquid hydrolysate, and washed filtrate.
To compare different pretreatment conditions in a normalized manner, the severity factor was calculated using Equation (1) (Overend et al., 1987):
where t is the residence time in minutes, T is the reaction temperature (°C), and 14.75 is the convention activation energy assuming that the overall reaction is hydrolytic reaction and the overall conversion is first-order reaction (Montane et al., 1998; Silverstein et al., 2007; Viridiana et al., 2010).
The severity factor evolved into a CSF to account for the addition of catalysts (Chum et al., 1990). Therefore, the CSF [Log(R′0)] described the severity of the pretreatment as factors of residence time, reaction temperature and pH, and was defined as follows Equations (2) and (3) (Pedersen and Meyer, 2010).
where the effect of an acid catalyst is taken into account by adding the final proton concentration in the pretreatment.
Enzymatic hydrolysis (EH) was performed to evaluate the efficiency of the organosolv pretreatment depending on temperature. The cellulase complex of NS 50013 (700 EGU/g, 1.2 g/mL) and β-glucosidase of NS 50010 (250 CBU/g, 1.2 g/mL) was kindly provided by Novozymes Korea (Seoul, Korea).
The solid residue, which was not lyophilized, was prepared at 1% (w/w) solid loading by mixing 1.0 g of dry weight with 100 mL of 50 mM sodium acetate buffer (pH 5.0) in a 250 mL Erlenmeyer flask. To the flask, 300 EGU/g dry biomass of NS 50013 was added and NS 50010 was supplemented at 30% of the weight of NS 50013. EH was performed at 50°C and 150 rpm for 72 h.
The hydrolysate was filtered through a 0.45-um hydrophilic PTFE syringe filter (Advantec). The glucose content in the hydrolysate was determined using high-performance liquid chromatography (HPLC; HP 1100 series, Agilent Technologies, Santa Clara, CA, USA) at the National Instrumentation Center for Environmental Management (NICEM, Seoul, Korea), using an Aminex HPX-87P column (300 mm × 7.8 mm ID × 9 um, Bio-Rad Laboratories, Richmond, CA, USA) and refractive index detector (RID). The efficiency of EH was calculated using Equations (4) and (5):
where the glucose content before EH was measured in the solid residue and the glucose content after EH was determined via HPLC.
The structural carbohydrates, lignin, total sugar (monomeric and oligomeric sugars), degradation products [5-hydroxymethylfurfural (5-HMF), furfural, and others], organic acids (levulinic acid, formic acid, and others), and other constituents were determined according to the NREL Laboratory Analytical Procedure (Sluiter et al., 2008a, 2008b).
The lyophilized solid (0.3 g) residue and 3.0 mL of 72% (w/w) sulfuric acid in an Erlenmeyer flask were hydrolyzed at 30°C for 60 min by stirring. The hydrolysate was diluted to a 4% concentration by adding 84.0 mL of distilled water and then autoclaved for 1 h at 121°C. The hydrolysate was cooled to room temperature and filtered through a previously weighed filter crucible (1G4, Iwaki, Tokyo, Japan). The residue was used for Klason lignin analysis and the filtrate was used for acid-soluble lignin (ASL) and structural carbohydrate analyses.
For Klason lignin analysis, the residue in the filtering crucible was dried at 105°C and then weighed. For ASL analysis, the absorbance of the filtrate was measured at 205 nm using a UV-visible spectrophotometer (UV-1601PC, Shimadzu, Kyoto, Japan). The ASL content was calculated using Equation (6):
where ε is absorptivity, equals to 110 L/g ∙ cm.
Liquid hydrolysate was filtered through a 0.45-um hydrophilic PTFE syringe filter and then analyzed via HPLC. The HPLC was equipped with an Aminex HPX-87H column and was operated at a column temperature of 40°C with a 0.5 mL/min flow of 0.01 N sulfuric acid as the mobile phase. The detectors used were an RID and a diode array detector at 210 and 284 nm, respectively. RID was used for quantitative analysis. Peaks were identified by comparing the peak retention times and UV absorbance patterns of the samples and standards. Calibration curves of the peaks were obtained using standard compounds at various concentrations and were used to determine the linear relationship between the peak area and concentration.
For the total sugar (monomeric and oligomeric sugars) content analysis, based on pH of the liquid hydrolysate, the amount of 72% (w/w) sulfuric acid required to bring the acid concentration of each aliquot to 4% was added into the liquid hydrolysate, and then the sealed sample was autoclaved for 1 h at 121°C. The autoclaved sample was neutralized to a pH of 5–6 by adding calcium carbonate.
For structural carbohydrate analysis, the filtrate was neutralized to a pH of 5–6 by adding calcium carbonate. After reaching pH 5–6, the autoclaved sample and filtrate were allowed to settle, and the clear liquid was decanted.
The clear liquid obtained from the autoclaved sample and filtrate, respectively, were filtered through a 0.45 um hydrophilic PTFE syringe filter and then analyzed via HPLC using an Aminex HPX-87P column. The HPLC was operated at a column temperature of 75°C with a 0.5 mL/min flow of HPLC-grade water as the mobile phase. A RID was also used.
The crystallinity index of the solid residue subjected to organosolv pretreatment and using Avicel PH-101 as a cellulose standard was measured using X-ray diffraction (XRD). XRD was performed on a powder XRD (D5005, Bruker, Karlsruhe, Germany) operated at 40 kV and 40 mA using CuKα (λ = 1.5406Å) radiation. The diffraction spectra were collected using θ–2θ method (Segal et al., 1954, 1959). The samples were scanned in duplicate at 1°/min from 2θ = 10°–30°, with a step size of 0.01°. The crystallinity index was calculated as the ratio of the area of crystalline contribution to the total area using the amorphous subtraction method (Park et al., 2010; Ruland, 1961).
Morphological changes on the surface of the lyophilized solid residue during organosolv pretreatment were analyzed using field-emission scanning electron microscopy (FE-SEM, SUPRA 55VP, Carl Zeiss, Oberkochen, Germany). The lyophilized solid residue was mounted on an aluminum stub using carbon tape with conductive silver paint applied to the sides to reduce sample charging and then sputter-coated with Pt-Pd using a sputter coater. The images were acquired at a beam voltage of 3 kV (Donohoe et al., 2008).
3. RESULTS and DISCUSSION
L. tulipifera contains 42.1% glucan, 20.7% xylan, 21.7% Klason lignin, 3.6% ASL, and other components (Table 1). The conversion characteristics of the major components in L. tulipifera were investigated depending on the temperature during the acid-catalyzed organosolv pretreatment. Pretreated L. tulipifera could be divided into three parts: solid residue, liquid hydrolysate, and precipitate [ethanol organosolv lignin (EOL)], as shown in Fig. 1.
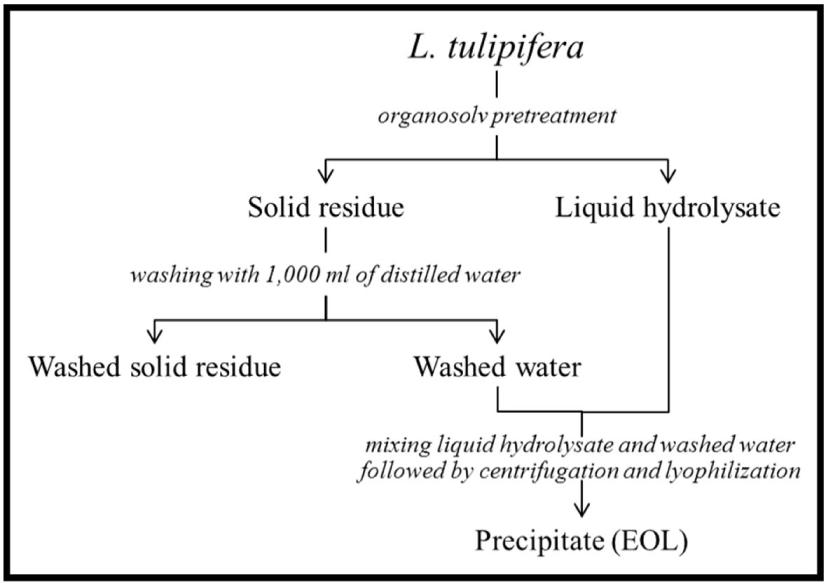
The CSF during the acid-catalyzed organosolv pretreatment is shown in Table 2. Because the pH was constant owing to the use of only 1.0% (w/w) sulfuric acid, the CSF varied depending on the temperature and residence time. As the temperature and residence time increased, the CSF increased continuously; however, a drastic increase in the CSF was not observed.
As shown in Fig. 2, as the temperature increased from 120°C to 180°C, the contents of xylan, Klason, and ASLs decreased; therefore, the content of glucan in the pretreated solid residue increased from 46.1% to 88.6%. At 180°C, xylan was completely hydrolyzed and converted, and only 10.4% of the Klason lignin and 1.0% of the acid soluble lignin remained in the pretreated solid residue.
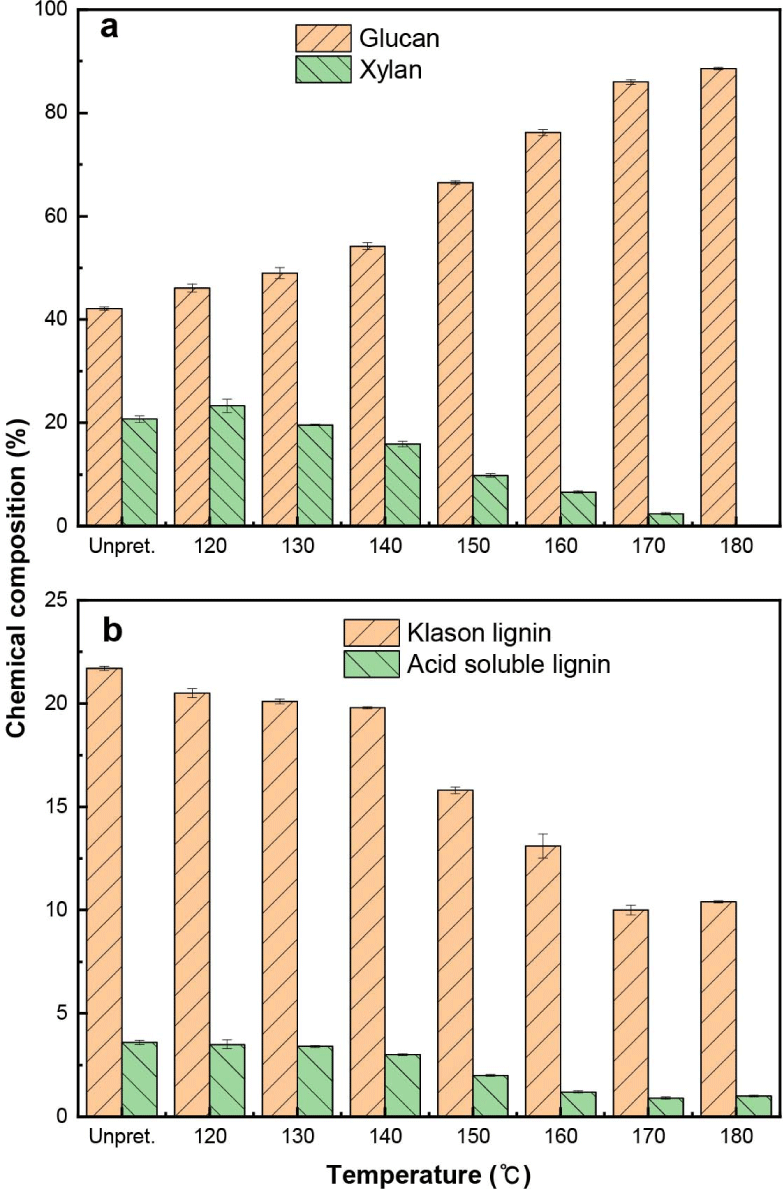
The residual ratio of each component in the pretreated L. tulipifera, depending on the temperature, was determined based on the initial content of each constituent in the non-pretreated L. tulipifera (Table 3). As the temperature increased, the water-insoluble solid residue of L. tulipifera decreased, indicating that the major components were hydrolyzed and converted. The hydrolysis ratio of glucan was the lowest among the major components after organosolv pretreatment. As the temperature increased, glucan was slowly hydrolyzed and converted, and then at 180°C, 84.1% of the glucan remained.
However, the hydrolysis and conversion of xylan progressed rapidly and the xylan was completely hydrolyzed and converted at 180°C. Furthermore, Klason and ASLs were rapidly degraded, and at 180°C, only 19.1% of the Klason lignin and 11.4% of the ASL remained. Interestingly, in the temperature range of 140°C–150°C, xylan, Klason, and acid soluble lignin were drastically hydrolyzed and degraded, except for glucan. Because hemicellulose (xylan) and lignin in lignocellulosic biomass comprise the lignin-carbohydrate complex via ether and ester bonds, both xylan and lignin in L. tulipifera are simultaneously hydrolyzed and degraded in the same manner (Salvi et al., 2010). As xylan hydrolysis increased rapidly, lignin degradation increased simultaneously. However, as the temperature increased up to 180°C, xylose was completely hydrolyzed, while lignin that remained was not completely degraded after the complete hydrolysis of xylan. This means that although organosolv pretreatment was more effective for the pretreatment of lignocellulosic biomass with high lignin content, owing to the solubilization of lignin and the breakdown of internal lignin and hemicellulose bonds, solubilization was difficult when only lignin remained in the solid residue without the cleavage of xylan and lignin bonds.
Based on the initial glucan and xylan contents in non-pretreated L. tulipifera, the yields of each constituent in the liquid hydrolysate pretreated with L. tulipifera are shown as a function of temperature in Figs. 3 and 4, respectively. The glucan in L. tulipifera was hydrolyzed and converted into monomeric and oligomeric forms of glucose and 5-HMF, respectively (Fig. 3). As the temperature increased, the yield of the oligomeric form of glucose slowly decreased, whereas those of the monomeric form and total glucose increased. Over 170°C, the total glucose yields rapidly increased as a result of the rapid hydrolysis of glucan in L. tulipifera (Table 3). To understand the conversion characteristics of glucan and xylan, comparisons were made with the hydrolysis and conversion of cellulose and xylan standards (Gwak et al., 2022).
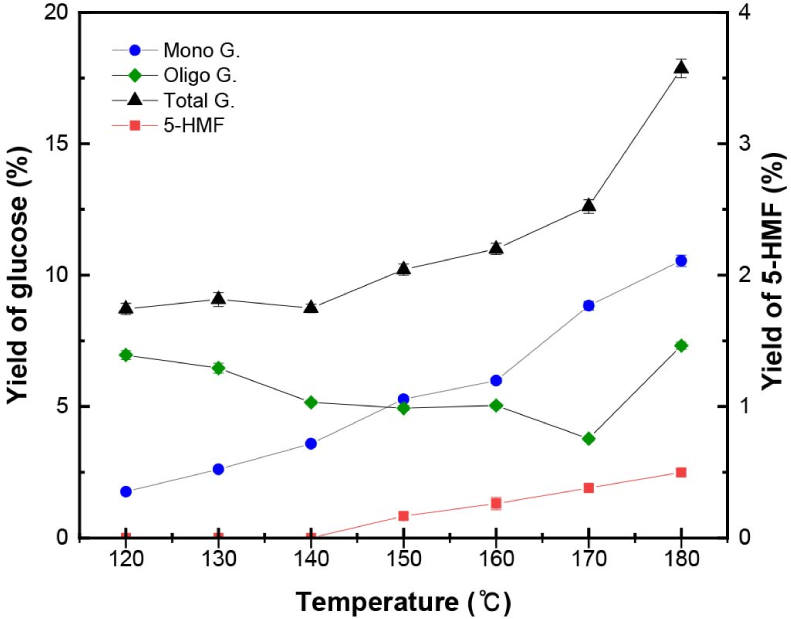
5-HMF was the major product produced from glucose, which began to be formed at 150°C. As the temperature increased, the yield of 5-HMF slightly increased, but at 180°C, its yield was no more than 0.4%. This value was similar to the yield of 5-HMF from the cellulose standards at 180°C and it was supposed that the yield of 5-HMF was affected by the temperature.
In this study, levulinic and formic acids were not formed during organosolv pretreatment of L. tulipifera (Fig. 3) in the range of 120°C–180°C. In cellulose standards, levulinic acid and formic acid began to be formed at 190°C; therefore, it seemed that levulinic and formic acids were formed at higher temperature and affected by the temperature.
As shown in Fig. 4, the xylan in L. tulipifera was hydrolyzed and converted into monomeric and oligomeric forms of xylose, acetic acid, and furfural. Acetic acid and furfural were the major decomposition products of xylan in L. tulipifera. As the temperature increased from 120°C to 140°C, the yield of xylooligomer was higher than that of the monomeric form of xylose, but the formation of the xylose monomer was accelerated in the range of 140°C–150°C, and consequently, the total xylose yield rapidly increased due to the drastic hydrolysis of xylan in L. tulipifera, as mentioned in section 3.1.1. Around 170°C, the yield of the monomeric form of xylose decreased, and this trend was the same as that of the xylose standard.
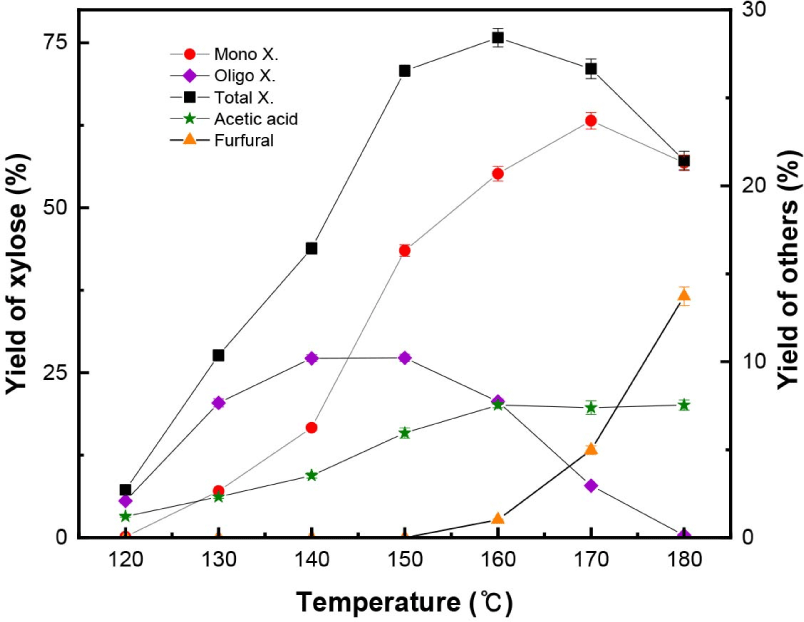
Xylan in lignocellulosic biomass contains approximately seven O-acetyl groups per ten xylose units; therefore, during acid hydrolysis, acetyl groups are hydrolyzed into acetic acid (Sjöström, 1993). In this study, acetic acid was formed at a lower temperature (120°C) than furfural. As the temperature increased, the yield of acetic acid increased, but over 160°C, its content remained at 6.5%–6.6%. It seemed that because most xylan was completely hydrolyzed over 160°C, no more acetic acid was formed in the range of 160°C–180°C. In the case of the xylose and xylan standards, acetic acid was not formed because of the absence of acetyl residues.
Furfural began to be formed at 160°C, and as the temperature increased up to 180°C, its yield increased to 12.1%. Although over 180°C, furfural was formed from the cellulose standards, its yield was very low. Therefore, it can be inferred that furfural was formed from xylan during the organosolv pretreatment of L. tulipifera. Additionally, furfural from the xylan standard began to be formed at 140°C, while furfural from xylan in L. tulipifera began to be formed at 160°C.
A precipitate, defined as EOL, can be obtained by mixing the liquid hydrolysate and washed water, primarily containing soluble lignin and monomeric and oligomeric hemicelluloses (Hallac et al., 2010). During the organosolv pretreatment of L. tulipifera, the precipitate contained more than 90% Klason and ASLs. At certain temperatures, small amounts of glucose and xylose were also present (Table 4).
Both xylan and lignin in L. tulipifera were hydrolyzed and degraded in the temperature range of 120°C–140°C, while the yield of the precipitate increased, and along with Klason and acid soluble lignins, a small amount of glucose and xylose were found in the precipitate (Table 4). As the temperature increased from 140°C to 150°C, the precipitate yield drastically increased because of the drastic hydrolysis and degradation of xylan and lignin into the liquid hydrolysate. In this range, it was assumed that the lignin was degraded into bulk size or small fractions, followed by self-condensation. Above 150°C, the precipitate yield slowly increased, and the xylose content in the precipitate disappeared at 170°C (Table 5). It was theorized that the formation of an additional precipitate from lignin degradation rapidly decreased, and the precipitate that had already formed from lignin began to rapidly and regularly degrade into smaller fractions without condensation (Nair et al., 2023). As described in section 3.1.1., because of the lignin-carbohydrate complex of L. tulipifera, both xylan and lignin were simultaneously hydrolyzed and degraded into liquid hydrolysate. However, after the complete hydrolysis of xylan, the degradation of lignin and its solubilization into the liquid hydrolysate almost did not progress, resulting in a constant yield of the precipitate at higher temperatures. Furthermore, during the simultaneous hydrolysis and degradation of xylan and lignin, the breakdown of internal lignin and xylan bonds and lignin solubilization occurred simultaneously, representing the characteristic conversions of organosolv pretreatment.
During organosolv pretreatment, monosaccharides (glucose and xylose), polysaccharides (glucan and xylan), and lignins (Klason and ASLs) undergo characteristic conversions in the solid residue, liquid hydrolysate, and precipitate states, depending on the temperature. Therefore, the influences on the structural and accessibility properties of the solid residue and the relationships between the characteristic conversions and structural and accessibility properties were investigated.
The crystallinity index is affected not only by the crystalline region of cellulose, but also by the amorphous regions of cellulose, hemicellulose, and lignin. As shown in Fig. 5, as the temperature increased from 120°C to 180°C, the crystallinity index continuously increased. The increase in the crystallinity index resulted from the decrease in amorphous regions caused by the hydrolysis and degradation of xylan and lignin and the hydrolysis of amorphous regions in glucan. Interestingly, in the range of 140°C–150°C, the crystallinity index was constant, although amorphous regions were drastically hydrolyzed and degraded. These results suggest that, in this range, critical hydrolysis of the crystalline regions in glucan might also occur.
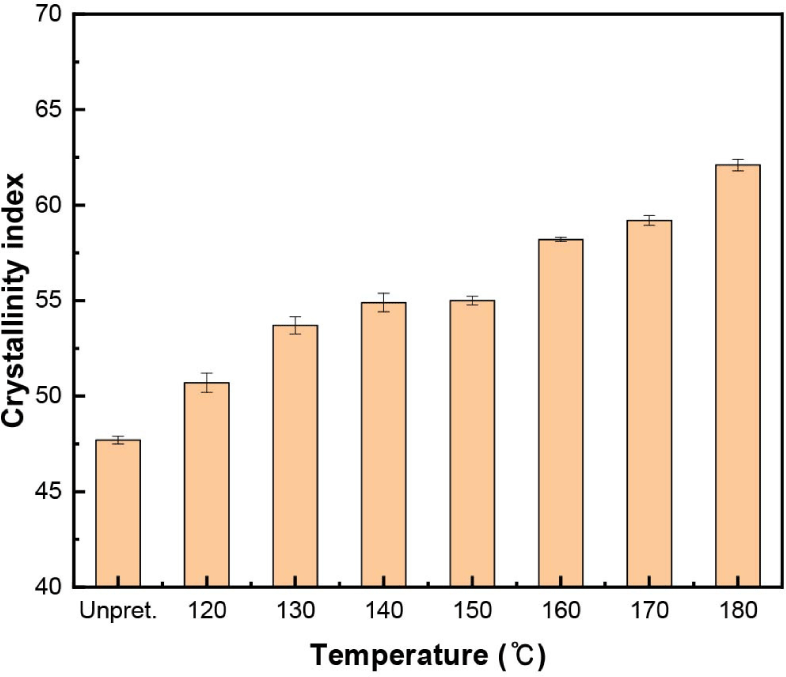
Compared with Avicel PH-101, as the temperature increased, the crystallinity index of Avicel PH-101 decreased slightly from 67.9% to 61.9% (Fig. 6), but the crystallinity index of the pretreated solid residue continuously increased from 47.7% to 62.1% (Fig. 5). At 180°C, the crystallinity index of the pretreated solid residue was similar to that of Avicel PH-101. Because Avicel PH-101 is mainly composed of microcrystalline cellulose, its crystalline regions were only hydrolyzed and degraded during organosolv pretreatment; therefore, its crystallinity index decreased. However, in the pretreated solid residue, glucan, which represents the main crystalline region, was slowly hydrolyzed, while the amorphous regions were rapidly hydrolyzed and degraded. Therefore, the crystallinity index of the solid residue increased.
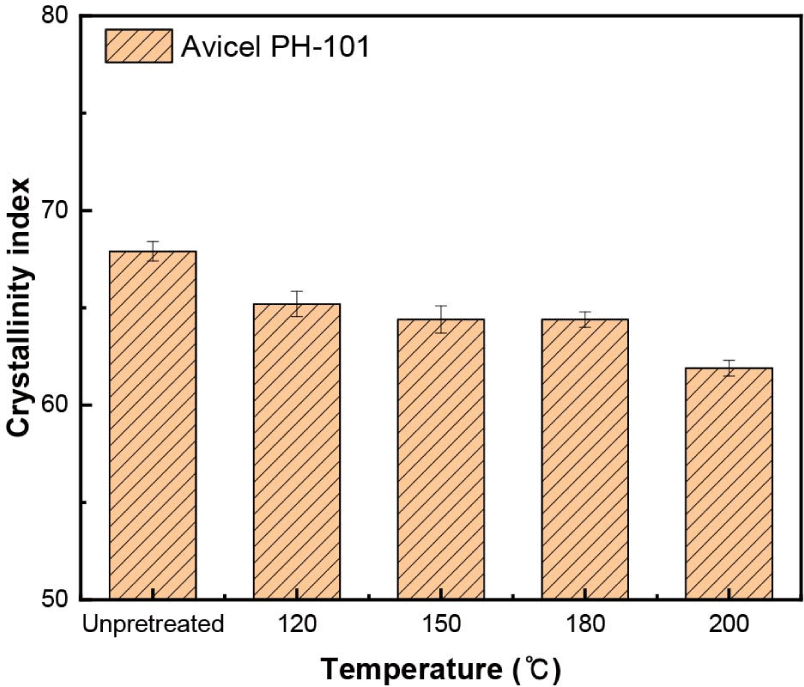
FE-SEM was used to investigate the morphological changes on the surface of the pretreated solid residue during organosolv pretreatment. The unpretreated L. tulipifera had a sleek, smooth surface; however, as the temperature increased, the surface became rough, and the formation of non-spherical or spherical droplets increased (Fig. 7). Over 160°C, the number of droplets drastically increased, and the droplets were coalesced into larger bodies (Fig. 8).
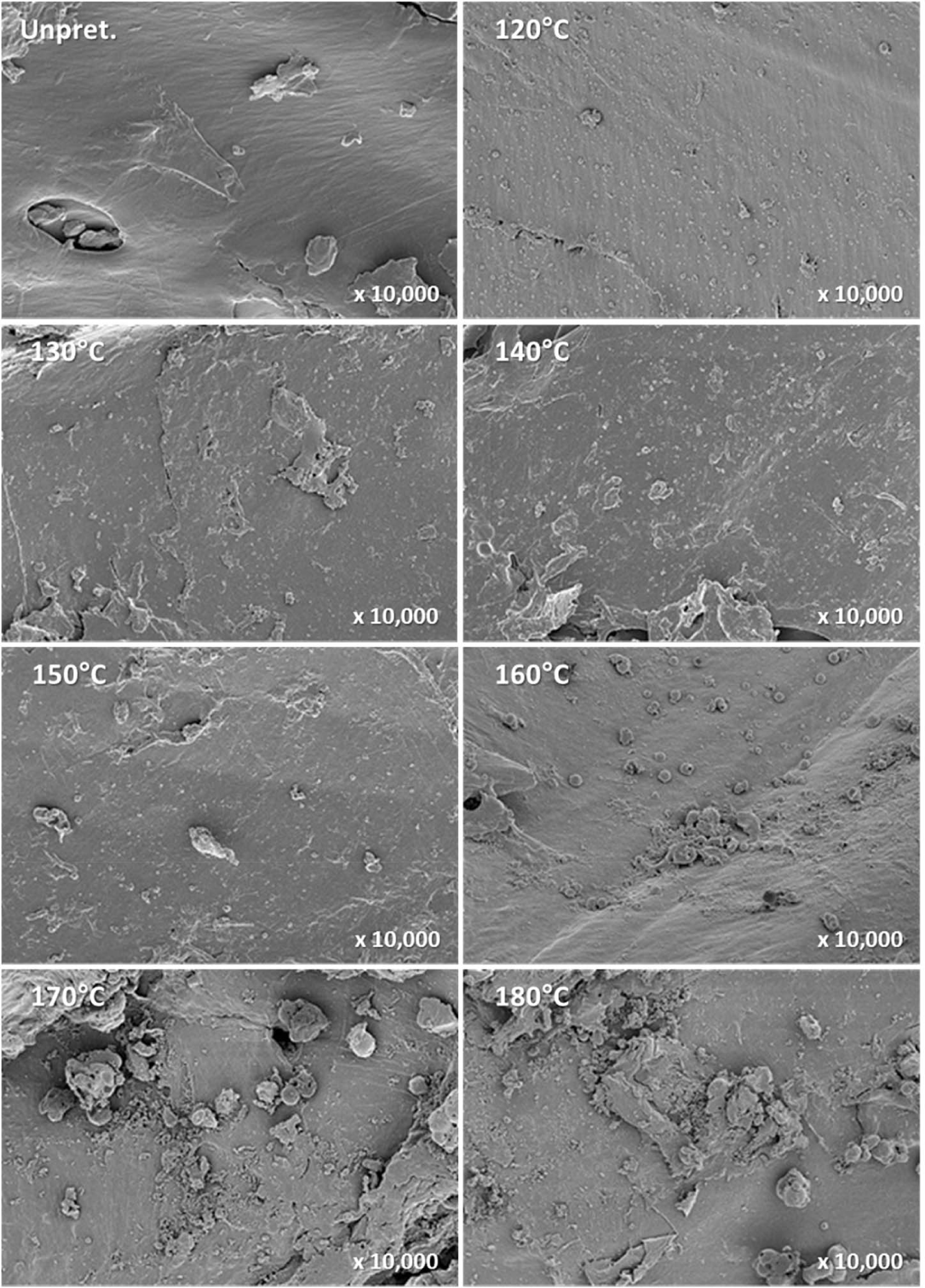
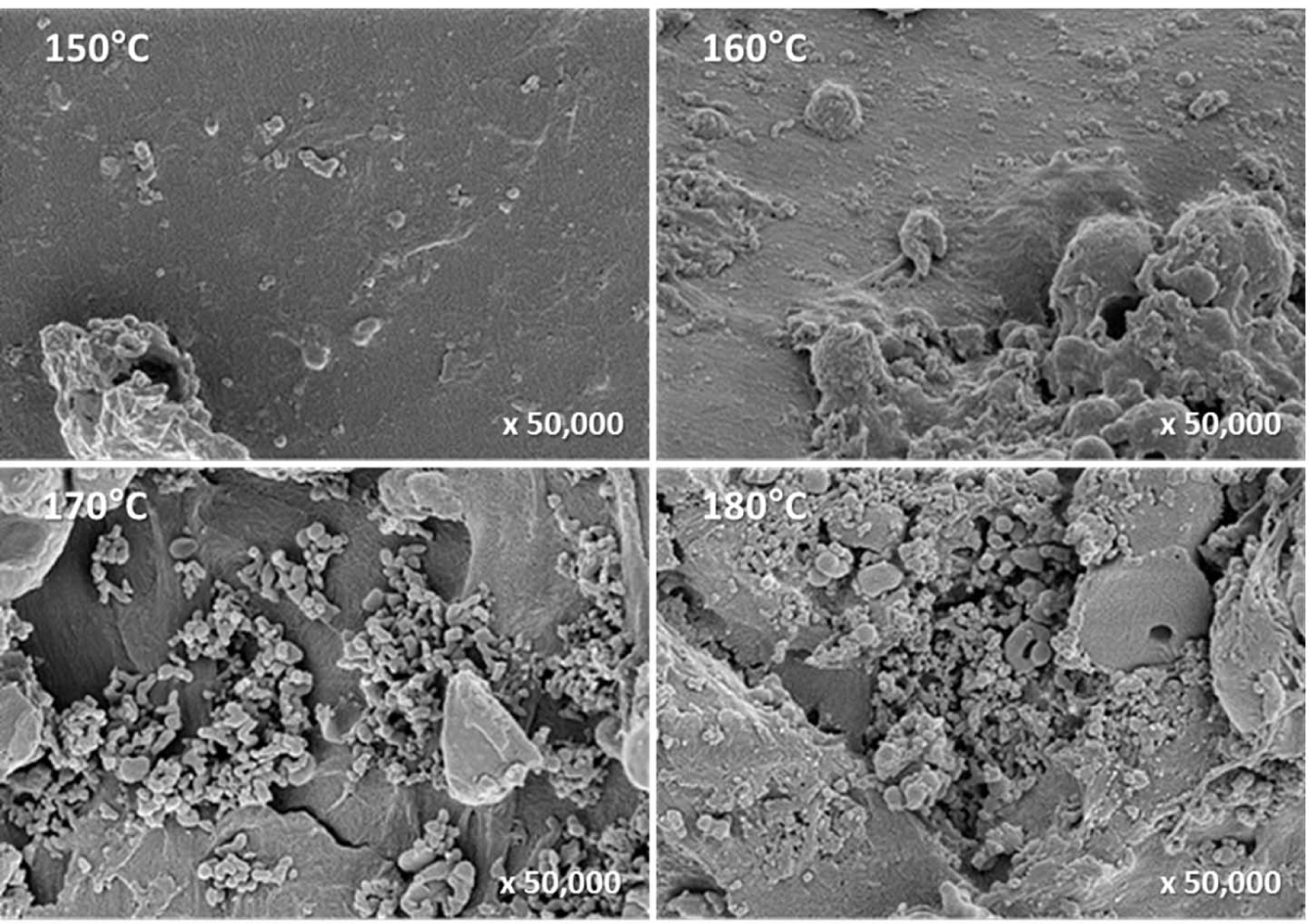
It has been reported that under various thermochemical pretreatments above the temperature of the lignin phase transition, lignin coalesces into a larger size, migrates within and out of the cell wall, and is redeposited on the surface of the cell wall. Therefore, lignin droplets were formed and observed during this process (Donohoe et al., 2008). The lignin droplets exhibited various morphological differences in terms of size, shape, surface texture, and density. Furthermore, the lignin droplets formed by hot water pretreatment at 220°C were predominantly composed of lignin and not of the lignin-carbohydrate complex.
The droplets formed during organosolv pretreatment in this study were considered to be the lignin droplets reported in previous studies because their shape, distribution, and surface were similar to those of the lignin droplets (Donohoe et al., 2008).
In the range of 120°C–150°C, both xylan and lignin in L. tulipifera were rapidly hydrolyzed and degraded into liquid hydrolysate (Table 3), but the drastic change related to lignin droplets was not observed. It is likely that in this temperature range, the non-spherical lignin droplets that formed and were deposited on the surface might contain not only lignin but also the lignin-carbohydrate complex. However, the chemical composition of the droplets could not be determined. In the range of 160°C–180°C, the xylan that remained was completely hydrolyzed and degraded into liquid hydrolysate, while the lignin that remained was not completely solubilized into liquid hydrolysate (Table 3). Therefore, lignin that was not solubilized, coalesced, migrated, and was redeposited on the surface of the solid residue (Fig. 8). Consequently, the formation and number of lignin droplets significantly increased. It is likely that at 180°C, the lignin droplets were predominantly composed of lignin, owing to the complete hydrolysis of xylan.
Through FE-SEM, the characteristic morphological changes related to lignin droplets were investigated during the organosolv pretreatment of L. tulipifera. However, although the drastic hydrolysis and degradation of xylan and lignin in L. tulipifera occurred in the range of 140°C–150°C, drastic morphological changes on the pretreated solid residue were not observed. In this range, lignin removal is preferred over the coalescence, migration, and redeposition of lignin.
EH was performed to evaluate the influence of the results obtained from the conversion characteristics of the major components and the structural properties of the solid residue on lignocellulosic biomass recalcitrance. The efficiency of EH was evaluated based on the cellulose-to-glucose conversion yield and enzymatic digestibility (Koo et al., 2011).
As the temperature increased, both the cellulose-to-glucose conversion yield and enzymatic digestibility continuously increased (Fig. 9). After EH of the solid residue that was pretreated over 150°C, the glucan that remained in the solid residue was almost completely hydrolyzed into glucose [Fig. 9(a)]. From 100 g of initial L. tulipifera, 43.8–44.0 g of glucose was obtained in the range of 150°C–160°C after organosolv pretreatment followed by EH (Table 6). Although the cellulose to glucose conversion yield reached 100% in the range of 170°C–180°C, the glucose content obtained from EH decreased due to the decrease in initial glucose content in the pretreated solid residue as a result of the increasing temperature. As the temperature increased from 140°C to 150°C, both the cellulose to glucose conversion yield and enzymatic digestibility drastically increased. Therefore, this temperature range is considered the critical pretreatment condition for effective improvement of EH by overcoming biomass recalcitrance.
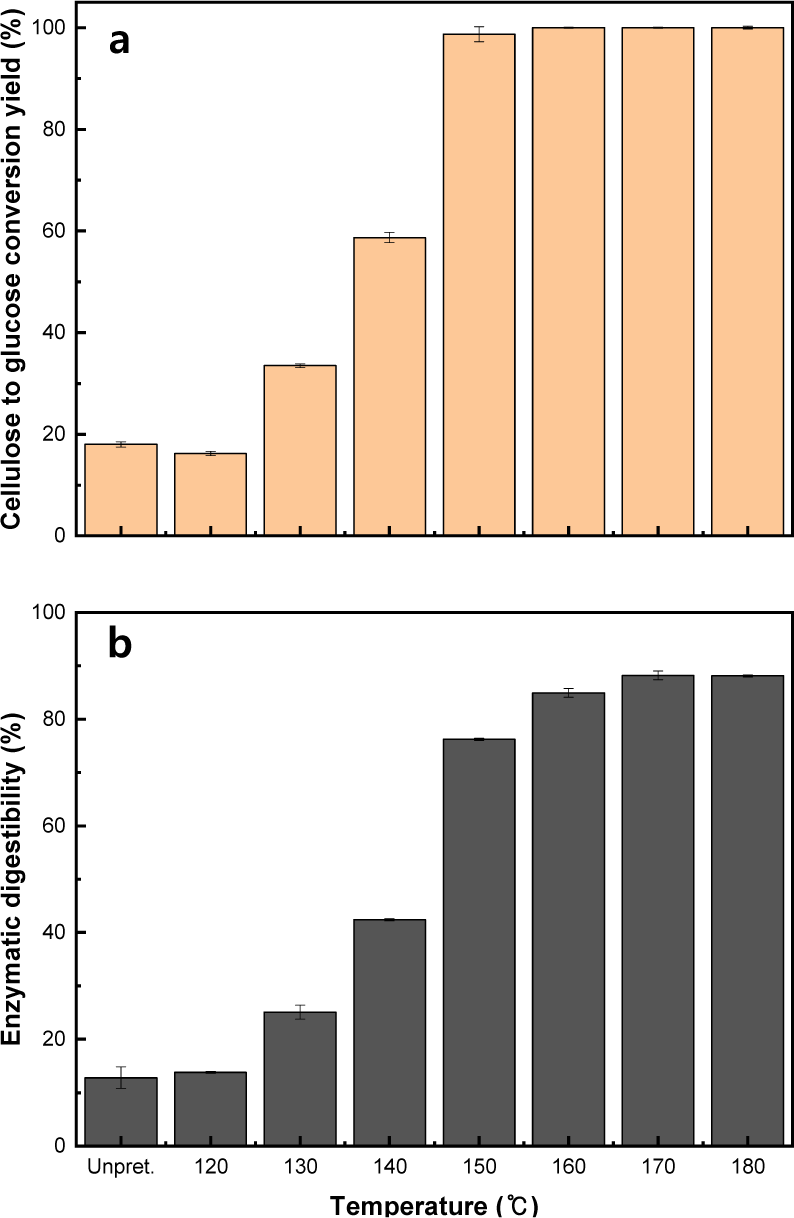
As a result of the conversion characteristics of the major components in L. tulipifera and their influence on the structural properties of the solid residue previously described in section 3.2.1., drastic characteristic changes were observed in the critical temperature range. These changes can be summarized as follows: a simultaneous decrease in xylan and lignin content, followed by the destruction of the lignin-carbohydrate complex, distribution of lignin, and deacetylation of hemicellulose. These characteristic changes resulted from the conversion of major components, such as glucose, xylose, glucan, xylan, and lignin, under specific conditions of organosolv pretreatment, and these conversion characteristics influenced the structural properties of L. tulipifera. Consequently, as mentioned in the introduction, these changes are considered very important factors in overcoming biomass recalcitrance during organosolv pretreatment, in addition to effectively improving the EH of lignocellulosic biomass.
4. CONCLUSIONS
During the organosolv pretreatment of L. tulipifera, glucan was slowly hydrolyzed, while other major components, such as xylan and lignin, were rapidly hydrolyzed. As a result of these conversion characteristics of organosolv pretreatment, the hydrolysis and degradation of xylan and lignin occurred simultaneously; however, after complete hydrolysis of xylan, the remaining lignin was not completely degraded and solubilized into the liquid hydrolysate. The other decomposition products during the organosolv pretreatment of L. tulipifera were 5-HMF formed from glucan, acetic acid, and furfural from xylan. Along with the solid residue and liquid hydrolysate, a precipitate containing more than 90% of lignin was formed.
In addition to the conversion characteristics of the major components of L. tulipifera, their influence of the conversion characteristics on the crystallinity and morphology of the solid residue was investigated. XRD analysis of the solid residue revealed that glucan, the main crystalline region, was slowly hydrolyzed as the temperature increased, whereas the amorphous regions were rapidly degraded; therefore, the crystallinity index increased. The presence and characteristic behavior of the lignin droplets were confirmed via FE-SEM analysis. These conversion characteristics considerably improve the efficiency of EH, such as the cellulose-to-glucose conversion yield and enzymatic accessibility.
The conversion characteristics of the major components of L. tulipifera are closely linked to the structural properties of the materials subjected to organosolv pretreatment and lignocellulosic biomass recalcitrance. Therefore, the results of this study are expected to support the effective utilization of lignocellulosic biomass in biorefinery processes using acid-catalyzed organosolv pretreatment.