1. INTRODUCTION
Cellulose nanofibrils (CNFs) are fibrous structures with a diameter of 100 nm or less that can be obtained from lignocellulose via a combination chemical pretreatment and mechanical treatment (Jang et al., 2014, 2017; Lee et al., 2011; Park et al., 2015). CNFs can be classified into three types depending on the chemical composition: Lignocellulose nanofibrils (LCNFs) containing cellulose, hemicellulose, and lignin; holocellulose nanofibrils (HCNFs) containing cellulose and hemicellulose; and pure cellulose nanofibrils (PCNFs) containing only cellulose (Han et al., 2017). Compared with HCNFs and PCNFs, the isolation of LCNFs requires less energy and has a lower environmental impact, as it does not require complete bleaching. Furthermore, the presence of lignin has been established to enhance the hydrophobicity and thermal stability of CNFs. CNFs have multiple beneficial characteristics, including eco-friendliness, non-toxicity, high transparency, high surface area, and excellent mechanical properties (Abe and Yano, 2012; Ji et al., 2017; Nakagaito and Yano, 2004, 2005; Okahisa et al., 2009), and have accordingly been applied in diverse fields as nanocomposite materials, in food packaging, and as components of drug delivery systems (Chen et al., 2019; Henriksson and Berglund et al., 2007; Kolakovic et al., 2012; Lee et al., 2019; Mishra et al., 2018; Park and Park, 2021; Yu et al., 2019; Zaini et al., 2019).
Given the recalcitrance of lignocellulose, isolation of CNFs is generally difficult and accordingly necessitate initial pretreatment (Han et al., 2020a; Zendrato et al., 2021). However, although a range of chemical pretreatments are available, most of these suffer from the drawback of using environmentally toxic reagents. Hence, there is a necessity to develop novel pretreatment methods that are less harmful to the environment. Among such green methods, enzymatic hydrolysis has been demonstrated to be effective for the preparation of CNFs. Endoglucanases, which are a class of cellulases that hydrolyzes cellulose, can facilitate the preparation of CNFs, based on their ability to degrade the β-1,4-glycosidic linkages of the amorphous regions of cellulose fibers (Henriksson et al., 2007). However, given the notably poor accessibility of untreated lignocellulose to these enzymes, initial pretreatment is essential to enhance enzyme accessibility (Fatriasari et al., 2020). In this regard, a readily preparable deep eutectic solvent (DES) has recently attracted attention as an effective green solvent for the pretreatment of lignocellulose (Francisco et al., 2012; Han et al., 2020b; Zhang et al., 2016). In addition, given that the enzymatic activity of cellulase enzymes does not necessitate the complete degradation of lignin, prolonged pretreatment using the DES is not necessary (Fu et al., 2010).
In this study, we developed a two-step process to prepare CNFs, in preliminary stage of which, DES pretreatment and enzymatic hydrolysis were performed on lignocellulose as an eco-friendly pretreatment, after which, CNFs were prepared via mechanical treatment. We also examined the effects of treatment conditions on the properties of the CNFs thus produced.
2. MATERIALS and METHODS
As source material for the preparation of CNFs, we used Pinus densiflora Siebold & Zucc. timber obtained from the Experimental Forest of Kangwon National University. Having prepared wood powder with 40–80 mesh size (180–425 μm), this material was extracted using ethanol/benzene (1:2, v/v) at 90°C for 8 h to remove extractives prior to DES treatment. The choline chloride (ChCl) and lactic acid (LA) used to prepare the DES were obtained from Daejung Chemical & Metals (Siheung, Korea), and the Acremonium cellulase used for hydrolysis was obtained from Meiji Seika (Tokyo, Japan).
DES was prepared by mixing ChCl and LA in a 1:1 molar ratio, followed by heating with stirring at 80°C until the solution was completely transparent. The lignocellulose was added to DES at a solid loading of 2 wt% and reacted at 130°C for 3 h with stirring. The reactant thus obtained was thereafter centrifuged twice at 10,000×g for 15 min. Having removed the DES soluble fraction (lignin-rich fraction), the DES insoluble fraction (polysaccharide-rich fraction) was washed with an excess of 1,4-dioxane/water (4:1, v/v), and finally washed with sodium acetate buffer (50 mM, pH 5.0) prior to enzymatic hydrolysis.
The lignin content of the lignocellulose thus obtained was measured using the Klason method. Having added 1 mL of 72% H2SO4 to 200 mg of lignocellulose and stirred for 2 h, the resulting mixture was diluted with 112 mL of distilled water to lower the concentration to 3%, which was followed by secondary hydrolysis in an autoclave at 120°C for 1 h. Following the reaction, the acid-insoluble fraction (Klason lignin) was collected by vacuum filtration using a glass filter and to remove residual acid, was washed with an excess of distilled water until obtaining a neutral pH.
Monosaccharide contents, including arabinose, galactose, glucose, xylose, and mannose, were determined using high-performance ion chromatography (Bio-LC, Agilent Technologies, Santa Clara, CA, USA) in conjunction with electrochemical detection using pulsed amperometry (gold electrode). The sample was chromatographed by passing through a CarboPac PA-1 column (Dionex, Sunnyvale, CA, USA), with the system being operated in isocratic mode at a flow rate of 1.0 mL/min with a mixture of 250 mM sodium hydroxide (20%) and deionized water (80%). The contents of glucose, corresponding to cellulose, and xylose, galactose, arabinose, and mannose, corresponding to hemicellulose, were calculated using the Chromeleon software program version 6.8 (Dionex). The contents of cellulose and hemicellulose were calculated based on these monosaccharide contents.
The enzyme activity of Acremonium cellulase was measured using the methods described by Ghose (1987) and Adney and Baker (2008). As a substrate, we used pieces of Whatman filter paper No.1 (1.0 cm × 6.0 cm in size), which were placed in test tubes and saturated with 1.0 mL of sodium acetate buffer (50 mM, pH 5.0) and 0.5 mL of different concentrations of enzyme solution. The mixtures were mixed for exactly the same time (10 s) using a vortex mixer and incubated at 50°C for 1 h. Reducing sugar was quantified using the DNS method (Miller, 1959). After incubation, 3 mL of DNS reagent was immediately added with continual mixing. Enzymatic hydrolysis was stopped by boiling at 96°C for 5 min and cooling down in ice–water. The sample was thereafter centrifuged to pellet the filter paper pulp and 200 μL of supernatant was extracted and diluted with 2.5 mL of deionized water. The absorbance of the prepare was determined spectrophotometrically at 540 nm and enzyme activity was calculated using the following Equation (1):
The numerator (0.918) in the equation is derived from the factor for converting the 5.0 mg of “glucose-equivalents” generated in the assay to millimoles of glucose (5.0/0.18016), from the volume of the enzyme being tested that is used in the assay (0.5 mL), and from the incubation time (60 min) required for the generation of the reducing equivalents.
DES-treated lignocellulose was diluted with sodium acetate buffer (50 mM, pH 5.0) to a concentration of 2 wt% and equilibrated at 50°C for 30 min prior to the addition of Acremonium cellulase at dosages of 1%, 3%, and 5% based on the dry weight of the DES-treated lignocellulose, and corresponding to 33.37, 100.11, and 166.85 FPU/g (filter paper unit, FPU) of DES-treated lignocellulose, respectively. Enzymatic hydrolysis was carried out at 50°C for 12, 24, and 48 h, with the reaction in each case being stopped by boiling the suspension at 96°C for 15 min. The reactant mixture was thereafter centrifuged at 15,000×g and the insoluble fraction was resuspended in distilled water at a concentration of 1.0 wt% and mixed using an HR-3752 high-speed blender (Koninklijke Philips, NV, USA) at 30,000 rpm for 15 min. The suspension was then uniformly dispersed to yield a 0.1 wt% preparation and subjected to five rounds of treatment using an MN400BF high-pressure homogenizer (Micronox, Sungnam, Korea). Fig. 1 shows a schematic representation of the LCNF preparation process. LCNFs obtained by DES treatment and by DES-enzyme treatment are labeled DES-LCNF and Enz-LCNF, respectively.
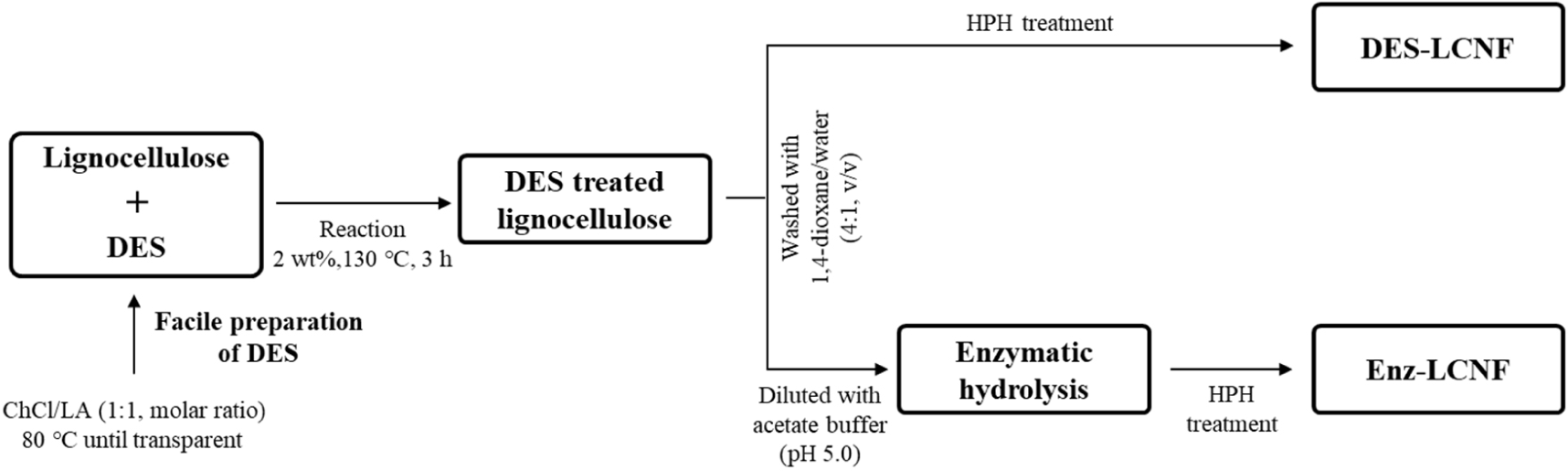
Sample suspensions with a solid content of 0.35 g were sonicated for 2 min and the dispersed sample was vacuum filtered using Whatman 1PS phase separator filter paper. Thereafter, the sheet was subjected to a pressure of 10 MPa for 5 min at 105°C using an HK-HP12T hand press device (Hankuk S & I, Hwaseong, Korea).
To examine the morphology of the prepared LCNFs, we performed scanning electron microscopy (SEM; S-4800, Hitachi, Tokyo, Japan) at an accelerating voltage of 1 kV. LCNF samples were prepared for SEM by sonicating a 0.001% suspension for 1 min using a VCX130PB ultrasonicator (Sonics & Materials, Newtown, CT, USA), with the sonicate subsequently being filtered using a PTFE membrane filter with a pore size of 0.2 μm. The filtration time was measured in this process. Following filtration, the preparation was immediately immersed in tert-butanol for 3 h, replacing the tert-butanol every 30 min, after which it was freeze-dried. The freeze-dried sample thus obtained was mounted on a metal stub using carbon tape and coated with iridium using a Leica EM ACE600 specimen coater (Leica, Vienna, Austria).
The water retention value (WRV) of the preparation was measured by initially placing a PTFE filter in a centrifuge tube containing a glass filter. The gel-state LCNF samples were placed on the PTFE filter and centrifuged at 2,000×g for 15 min using a swing rotor. The weight of the centrifuged cake-shaped sample was measured followed by drying at 105°C. WRV was calculated using the following Equation (2):
where Wc is the weight of the sample after centrifugation and Wo is the weight after oven drying.
X-ray diffraction (XRD) patterns of DES-LCNF and Enz-LCNF were obtained using an X’Pert PRO MPD X-ray diffractometer (Malvern Panalytical, Almelo, The Netherlands) in the range of 2θ = 5°–40°. The crystallinity index values of DES-LCNFs and Enz-LCNFs were calculated using the Segal Equation (3) as mentioned below.
where I002 and Iam are the intensities at 2θ = 22.7° and 18°, which represent crystalline and amorphous states, respectively.
Tensile strength was measured as follows. Specimens were prepared in dog bone shape of dimensions 3.0 mm × 0.02–0.04 mm × 6 cm (width × thickness × length) and retained at 25°C and 65% relative humidity to reduce the influence of temperature and relative humidity on tensile strength measurements. Tensile strength was measured using a TO-102D-100 kgf universal testing instrument (Test one, Siheung, Korea) at a cross head speed of 5 mm/min with a span length of 30 mm.
3. RESULTS and DISCUSSION
Fig. 2 and Table 1 show the chemical composition and monosaccharide contents of raw and DES-treated lignocelluloses. Lignin content was determined using the Klason method, whereas the contents of cellulose and hemicellulose were calculated based on the determinations of monosaccharide contents. The raw material was accordingly found to consists of 46% cellulose, 26% hemicellulose, and 28% lignin; whereas in comparison the DES-treated lignocellulose consisted of 38% cellulose, 2.5% hemicellulose, and 2.5% lignin, the contents of which were calculated based on the those of the raw material. Compared with that of cellulose, it can be seen that the contents of hemicellulose and lignin were substantially reduced by the DES treatment, thereby indicating that despite the short treatment time, the DES exhibits a remarkable selectivity for hemicellulose and lignin.
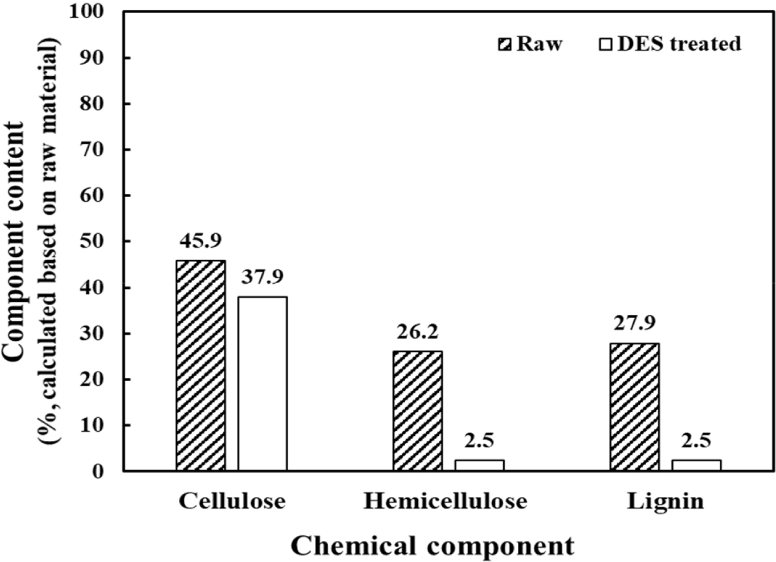
Sample | Monosaccharide content (%) | |||||
---|---|---|---|---|---|---|
Arabinose | Galactose Glucose | Xylose | Mannose | Total | ||
Raw material | 3.0 | 5.7 63.7 | 10.0 | 17.6 | 100.0 | |
DES-treated lignocellulose | 0.0 | 0.0 93.9 | 2.1 | 3.9 | 100.0 |
The glucose standard curve shown in Fig. 3(a) was constructed using the absolute amounts of glucose against absorbance at 540 nm. The amount of released glucose at each enzyme concentration was determined using the standard curve equation after subtracting the enzyme blank from the enzyme absorbance at 540 nm. Fig. 3(b) shows the formula describing the relationship between released glucose and enzyme concentration. As determined using this equation, the FPU activity of Acremonium cellulase was determined to be 166.87 FPU/mL, with one unit (U) of Acremonium cellulase activity corresponding to 1 mmol of glucose released per minute at pH 5.0 and 50°C.
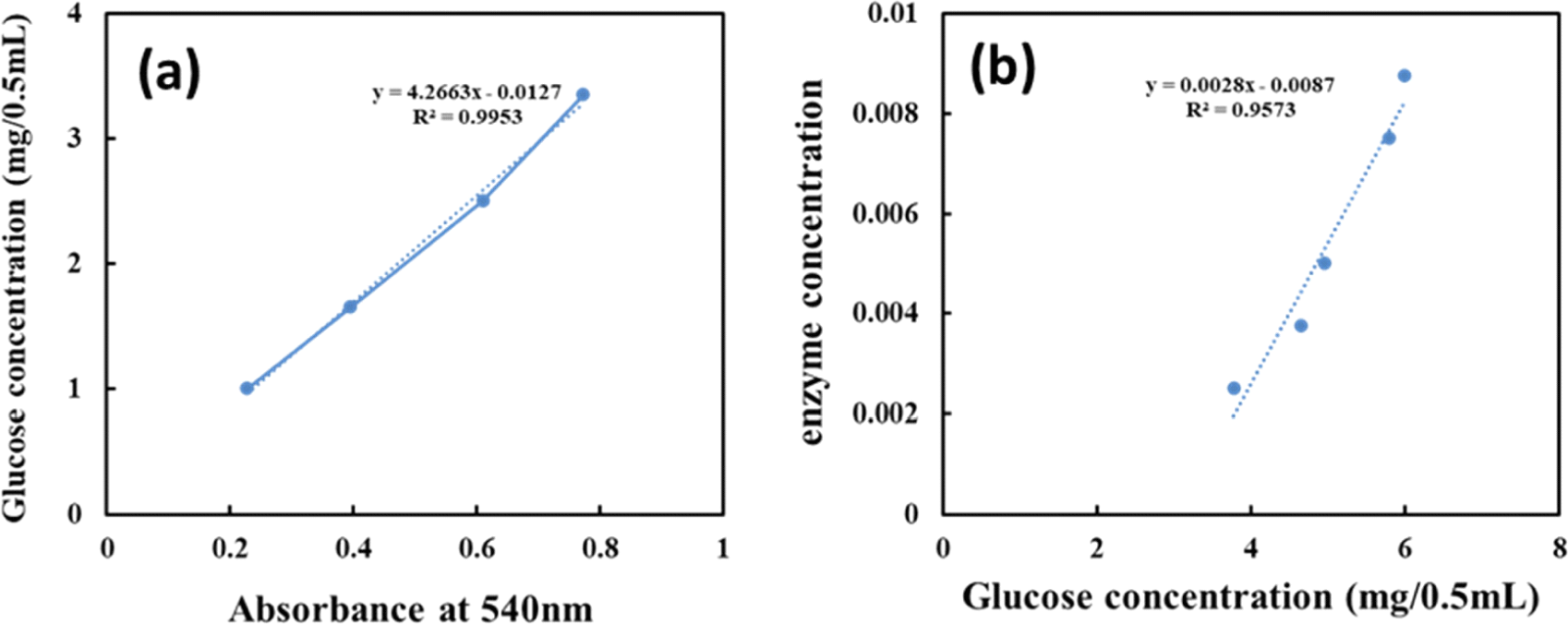
Morphological characteristics are important determinants of the nanoscale defibrillation of cellulose, and in Fig. 4, we show the morphologies of raw lignocellulose, DES-treated lignocellulose, DES-LCNFs, and Enz-LCNFs. Following DES treatment, the cellulose fibers were observed to be partially deconstructed, although no significant defibrillation was detected. In contrast, DES-LCNF and Enz-LCNF show very well defibrillated entangled web-like structures. Fig. 5 and Fig. 6 show the morphologies of DES-LCNFs and Enz-LCNFs produced under different enzymatic treatment conditions. Visually, we detected no significant differences in these materials, although the proportion of agglomeration was slightly reduced following enzymatic treatment. Well-dispersed homogeneous LCNFs were observed in all samples.
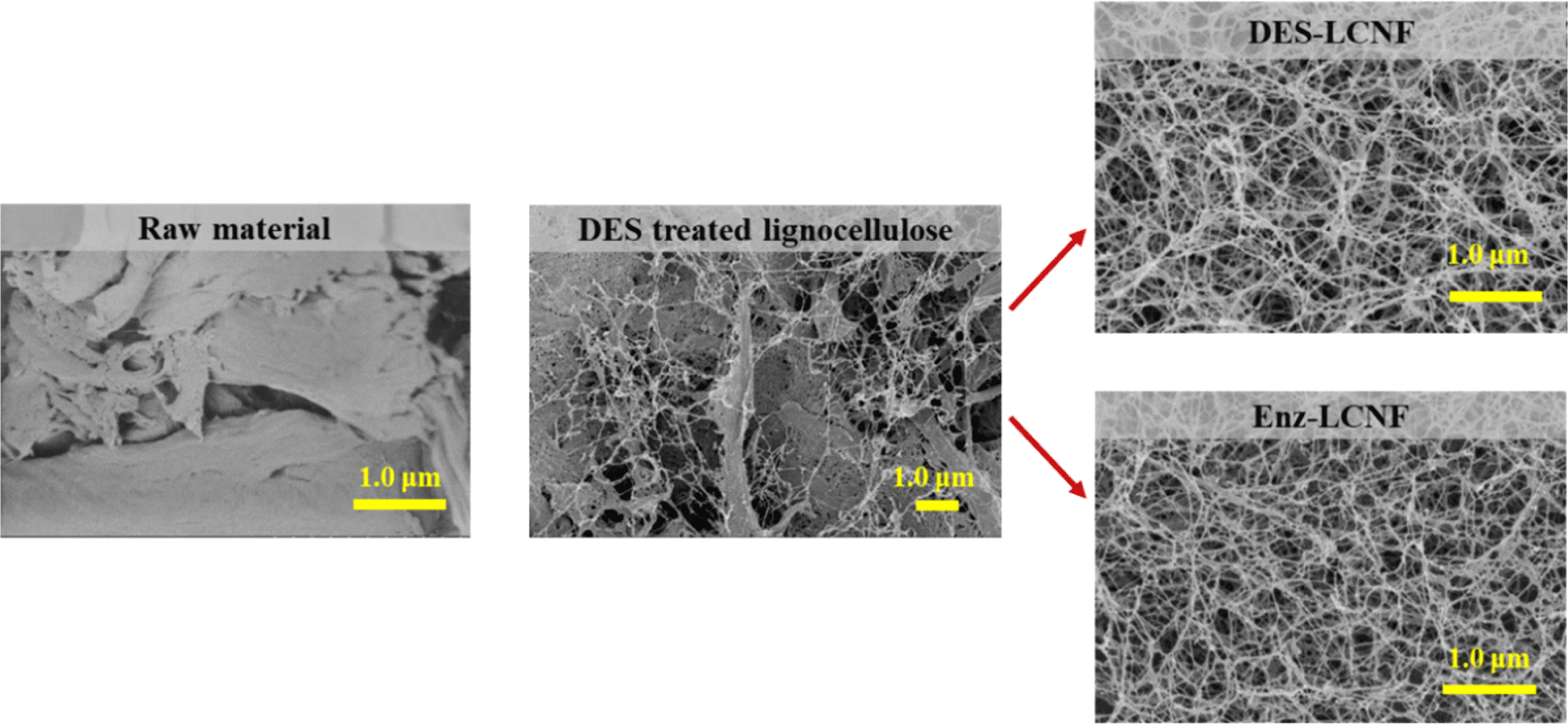
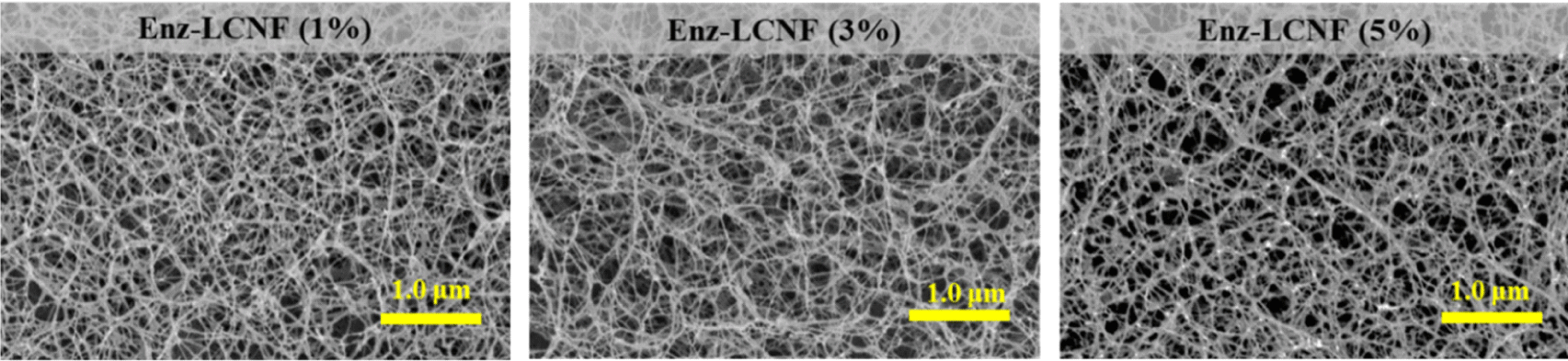
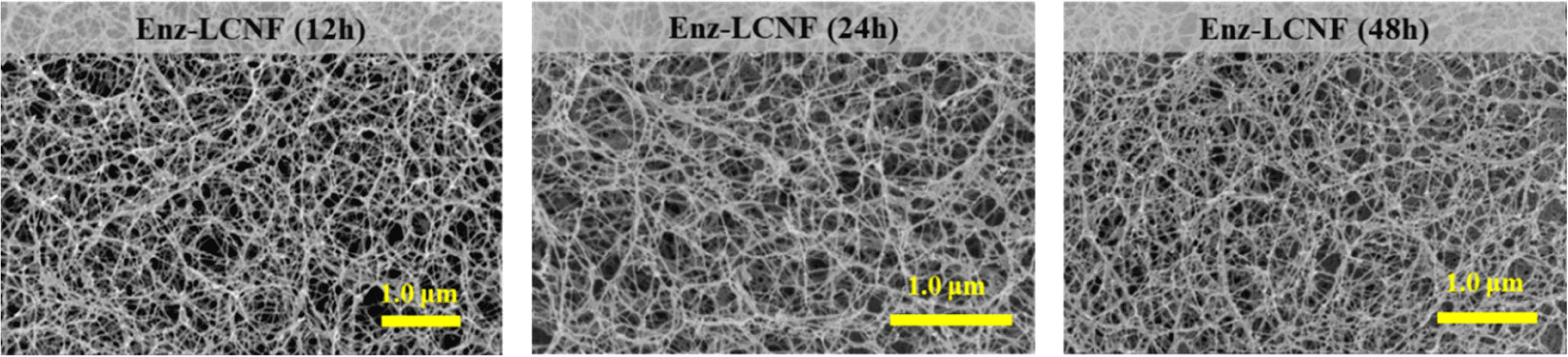
Table 2 shows the average diameter, filtration time, and WRV of DES-LCNFs and Enz-LCNFs. For all enzymatic treatment conditions, the average diameter of Enz-LCNFs was found to be smaller than that of DES-LCNFs (20.7 ± 4.0 nm). With an increase in the concentration of enzyme used during the preparation, we observed reductions in the average nanofibril diameter, filtration time, and WRV. Similarly, with an increase in enzymatic treatment time, there were reductions in the average nanofibril diameter and filtration time, whereas in contrast, WRV tended to increase. The smallest average diameter of the prepared LCNFs was 15.7 nm, obtained in 24 h treatments using an enzyme concentration of 5%, and filtration time was found to decrease as the enzyme concentration and treatment time increased. The water retention capacity of CNFs is indicative of hydrophilicity and the higher WRVs of CNFs compared with cellulose can be ascribed to an increase in specific surface area during the nanoscale defibration process, which is associated with an increase in the proportion of exposed hydroxyl groups (Song et al., 2019). Water retention tends to decline in response to an increase in enzyme concentration, whereas conversely, values generally increase as the enzymatic treatment time increases, albeit not substantially. In the present study, the highest WRV measured was 1,636.6%, which was obtained for CNFs prepared using an enzyme concentration of 3% for 48 h. Furthermore, WRV typically tends to increase as the degree of pretreatment increases and nano-sized fibrillation proceeds (Ryu et al., 2014; Song et al., 2019).
The effects of pretreatment on the crystallinity of CNFs are illustrated in the XRD patterns of DES-LCNFs and Enz-LCNFs presented in Fig. 7. All samples exhibited peaks corresponding to 2θ values of 7°, 15°, and 22°, which can be attributed to the typical structure of cellulose I. Enzymatic hydrolysis does not affect the typical cellulose I structure, which indicates that enzymatic hydrolysis at a certain concentration (1%) rarely causes damage to the crystalline form. Table 3 shows the CrI values of DES-LCNFs and Enz-LCNFs obtained under different treatment conditions. The crystallinity increases as the microfibrils are more densely bundled with increasing cellulose content and the crystallinity decreases as the microfibrils disperse with disintegration as CNFs (Daicho et al., 2018). The highest CrI was obtained for Enz-LCNF treated for 24 h using enzyme at a concentration of 1%. After treatment under these conditions, the CrI was higher than that LCNFs without enzyme treatment, which indicates that enzymatic treatment disrupts the amorphous region. The CrI of LCNF preparations was found to decrease with increases the enzyme concentration and treatment time, with the lowest value being recorded for Enz-LCNFs subjected to treatment with 5% enzyme for 24 h. We suspect that this reduction in CrI reflects the fact that the Acremonium cellulase used in this study has a greater cellobiohydrolase than endoglucanase activity.
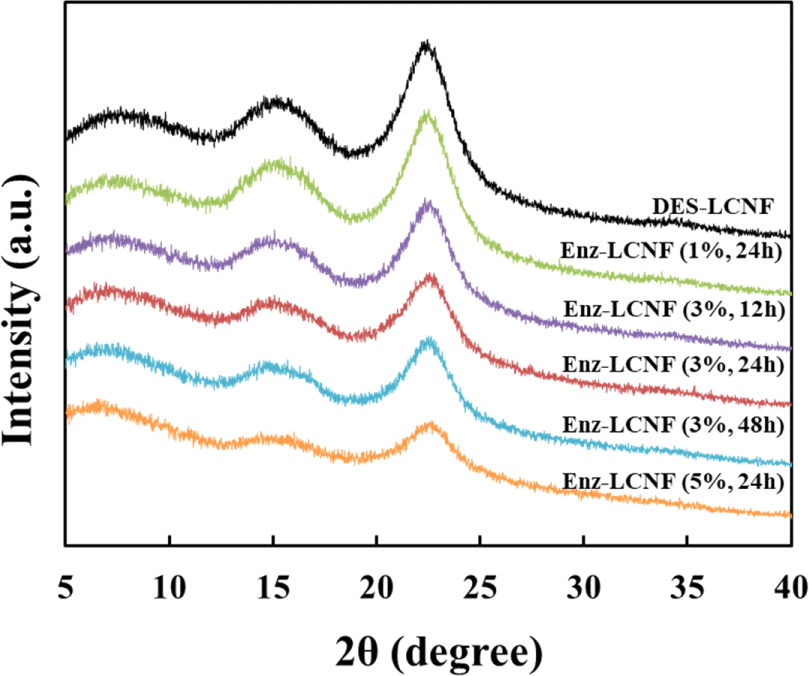
Sample | Enzyme concentration (%) | Enzyme treatment time (h) | CrI (%) |
---|---|---|---|
DES-LCNF | - | - | 44.9 |
Enz-LCNF | 1 | 24 | 45.5 |
3 | 12 | 40.8 | |
24 | 35.1 | ||
48 | 34.8 | ||
5 | 24 | 26.2 |
Table 4 shows the tensile strength, elastic modulus, and elongation at the break of DES-LCNF and Enz-LCNF nanosheets. We observed that the tensile strength of the Enz-LCNF nanosheets decreased in response to an increase in enzyme concentration and enzymatic treatment time. Compared with the DES-LCNF nanosheets, those of Enz-LCNFs tended to be characterized by lower tensile strength, with Enz-LCNF nanosheets showing the lowest mechanical properties when prepared using an enzyme concentration of 3% for 48 h. We also detected a reduction in the elastic modulus of Enz-LCNF nanosheets with an increase in enzyme concentration, although the trend was not pronounced, whereas values increased with a prolongation of the enzymatic treatment time. Moreover, we detected a reduction in the elongation at break with an increase in the enzyme concentration and enzymatic treatment time.
4. CONCLUSIONS
In this study, we devised a two-step DES-enzyme process for pretreatment of lignocellulose in the preparation of LCNFs, and evaluated the effects of enzymatic treatment conditions on Enz-LCNFs. The observed morphological characteristics of the Enz-LCNF products indicated a well-defibrillated entangled web-like structure. We found that the average diameter and filtration time of Enz-LCNFs tended to decrease in response to an increase in enzyme concentration and treatment time during enzymatic processing. Furthermore, we detected reductions in the water retention capacity of the obtained LCNFs as the enzyme concentration increases and enzymatic treatment time decreases. X-ray diffraction analysis revealed that both DES-LCNFs and Enz-LCNFs were characterized by a typical cellulose I structure, and we established that enzymatic treatment at a concentration of 1% rarely damaged the crystalline form. In addition, we found that an increase in the tensile strength of the produced LCNFs tended to be correlated with an increase in the crystallinity index. The tensile strength of Enz-LCNF sheets showed a tendency to decrease as the enzyme concentration and treatment time increased. The tensile strength of DES-LCNF sheet was generally higher than that of Enz-LCNF sheets, the highest value for the latter of which was obtained for sheets prepared under treatment conditions of 1% enzyme concentration for 24 h.