1. INTRODUCTION
Wood, a renewable resource and a natural polymer, is gaining increasing global attention as part of the collective effort to mitigate climate change. As one of the most abundant resources on the planet, wood has long been used for various applications such as interior and exterior construction material, household products, and fuel. Until recently, research has been conducted on fuel and exterior building materials such as CLT, plywood, MDF, etc (Fujimoto et al., 2021; Jang and Lee, 2019; Kang et al., 2019; Lee et al., 2019a; Yang et al., 2019). However, it is an organic composite and may deteriorate when exposed to outdoor environments owing to biological and non-biological factors. Wood undergoes discoloration and decomposition when exposed to light, i.e., photo-degradation; it also has a shortened service life when exposed to the outdoors for long durations (Borgin, 1971; Evans and Schmalzl, 1989; Kim and Kim, 2020; Kuo and Hu, 1991; Owen et al., 1993; Plackett et al., 1992; Tshabalala and Gangstad, 2003).
One of the major causes of the photo-degradation of wood is that lignin, one of its main components, absorbs UV light, resulting in the migration of radical intermediates and the subsequent formation of quinoid structures (Cogulet et al., 2016; Pandey and Vuorinen, 2008). Also, wood extractives that have double bonds and benzene rings in their structures undergo discoloration (Chang et al., 2010; Fan et al., 2009; Pandey, 2005). Many studies have explored ways to prevent this photo-degradation, among which the most widely used are wood-coatings to block or absorb UV light via UV stabilizers, absorbents, hindered amine light stabilizers (HALS), and quenchers. Most UV absorbents constitute organic matter; however, studies are actively exploring inorganic UV absorbents. Guo et al. (2016) and Lozhechnikova et al. (2017) coated objects with ZnO nanostructures, an inorganic material that can effectively absorb UV light. Pánek et al. (2017) reported wood-coating treatments using ZnO nanoparticles, hydrophobic materials, oils, and UV stabilizers. However, with coating treatments, there are issues such as the discoloration and durability of the coating; in case of UV stabilizers, they only treat the wood surface. Therefore, a simple but effective approach to control or remove the lignin components is essential to mitigate wood discoloration and the subsequent loss in quality.
Various researchers are investigating delignification methods using various solutions containing H2O2, NaClO2, NaClO, or Na2SO3 to control the lignin content of wood (Khanjanzadeh and Park, 2020; Lee et al., 2019b; Park et al., 2018a; Park et al., 2018b; Zendrato et al., 2021). Delignification methods that prevent wood photo-degradation can endow the wood with unique properties. Vasileva et al. (2017) at the Royal Swedish Institute of Technology reported a delignification treatment that used NaClO and subsequent polymer impregnation to create transparent wood, demonstrating the potential to create new materials. Kang and Lee (2005) reported improved sound absorption in delignified wood. Various studies have explored partial delignification of wood to alter its thermal conductivity and optical properties and to yield lightweight materials (Chen et al., 2020; Li et al., 2021). Delignification on various wood materials including wood flour are being conducted using chemicals that are used for bleaching in the paper industry to separate wood fibers.
Among the various solutions that are used for bleaching or delignification treatment, peracetic acid (PAA) has strong oxidizing ability and has been reported to promote the oxidation and decomposition of lignin at low temperatures, i.e., below 100°C, with relatively short treatment times (Kang et al., 2015; Ma et al., 2016; Park et al., 2019; Park et al., 2020a; Park et al., 2020b). Following previous studies, this study explored the delignification of wood with a stratified porous structure was conducted using PAA to prevent discoloration of the surface by selectively removing lignin. According to Kataoka (2008), the depth at which discoloration occurs due to degradation caused by weather or UV light does not exceed 34–2,540 μm; therefore, this study aims to use PAA to remove part of the lignin on the wood surface. To compare the extent of delignification among various wood species, the softwood Larix kaemferi and the hardwood Q. mongolica were employed as the test species. Also, hydrogen peroxide (HP) treatment was additionally conducted for comparison. After the surface chemical treatments, the samples were exposed to artificial UV light to induce photo-degradation, and the changes in the wood color, functional groups and surface images of the samples were observed.
2. MATERIALS and METHODS
L. kaempferi and Q. mongolica (18 years) were used for experimental specimens in this study. Woods were obtained from the academic forest of Kangwon National University in Hongcheon-gun, Gangwon-do, South Korea. L. kaempferi is a representative softwood for wooden building, and Q. mongolica is the most common hardwood species with the largest dominance in South Korea. Before experiment, wood specimens were prepared with dimensions of 30 (L) × 20 (R) × 40 (T) mm3 for each chemical treatment. These were conditioned in a climatic chamber at 24°C and 60% relative humidity. The images of the samples are listed in Fig. 1.
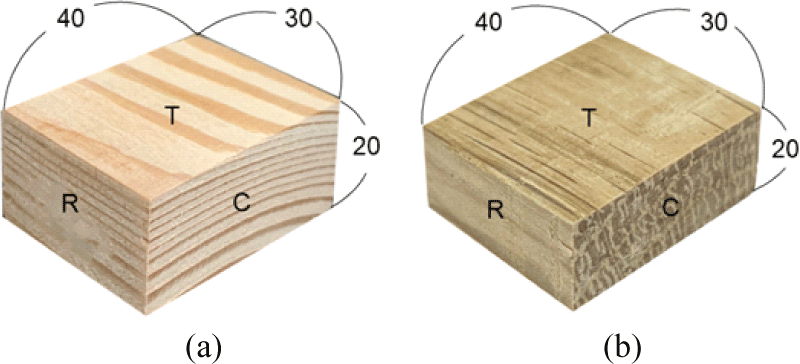
PAA and HP (30%, Daejung, Siheung, Korea) were used as solutions for the delignification treatment of the wood surface. In the case of PAA, it was prepared by mixing acetic acid (AA, 99%, Daejung) and HP in a ratio of 1:1.5 (v/v), and the concentration was titrated after storage at room temperature for 2 weeks in order to use the same concentration in the experiment. The concentrations of PAA was measured according to the concentration titration method reported in a previous study (Park et al., 2020a). On the other hand, in order to vary the concentration of the PAA solution, PAA and distilled water were mixed in a ratio of 1:1 (v/v). The concentration of PAA and HP are listed in Table 1. For the chemical treatment, 300 mL of each solution and 3 specimens were placed in a 1 L beaker and reacted at 70°C for 4, 8 h. After completion of the reaction, it was washed with distilled water and dried in a dark room at room temperature.
Condition | Abbreviation | PAA concentration (%) | HP concentration (%) |
---|---|---|---|
AA + HP (1:1.5, v/v) | P | 9.1 | 15.7 |
PAA + D.I water (1:1, v/v) | 1/2P | 5.8 | 7.9 |
HP only | HP | - | 30.0 |
After chemical treatment, weathering test was conducted to induce artificial photo-degradation on wood. Weathering conditions were set according to the ASTM G154; A xenon light at 0.89 W/m2, range of 340 nm, at 60°C, for 168 h in an accelerated weathering tester (QUV/se Accelerated Weathering Tester, Q-LAB, Westlake, OH, USA).
As shown in Fig. 2, the surface color of wood samples for 5 periods was measured using a colorimeter (CR-10 Plus, Konica Minolta, Tyoko, Japan). Color difference measurement were performed both before chemical treatment and after UV irradiation. According to the CIE Lab standard, the L*, a*, b* color coordinates were calculated based on three independent specimens at five different point. ΔL*, Δa*, and Δb* were the total changes of the L*, a*, and b* values during weathering testing, respectively. An increase/decrease in the L* value means the color of the sample becomes brighter/darker. A positive Δa* signifies a color shift toward red, and a negative Δa* signifies a color shift toward green. A positive Δb* signifies a shift toward yellow, and a negative Δb* signifies a shift toward blue. The color difference (ΔE) was following Equation (1). And The color difference can be categorized as shown in Table 2 (Hadi et al., 2022).
L0 – La, L0: initial L* values, La: final L* values.
a0 – aa, a0: initial a* values, aa: final a* values.
b0 – ba, b0: initial b* values, ba: final b* values.
Fourier transform infrared spectroscopy (FT-IR, Nicolet Summit, Thermo Fisher Scientific, Waltham, MA, USA) was used to observe the functional group changes of the specimens after chemical treatment of the wood surface and UV irradiation. The spectra were recorded in the wavenumber range from 800–2,000 cm−1 and 32 scans per sample with a resolution of 4 cm−1 were used.
3. RESULTS and DISCUSSION
Fig. 3 shows photographs of the wood surfaces to compare the color changes after the PAA and HP treatments at different concentrations and reaction times (4 h and 8 h) as well as the color differences after exposure to UV light.
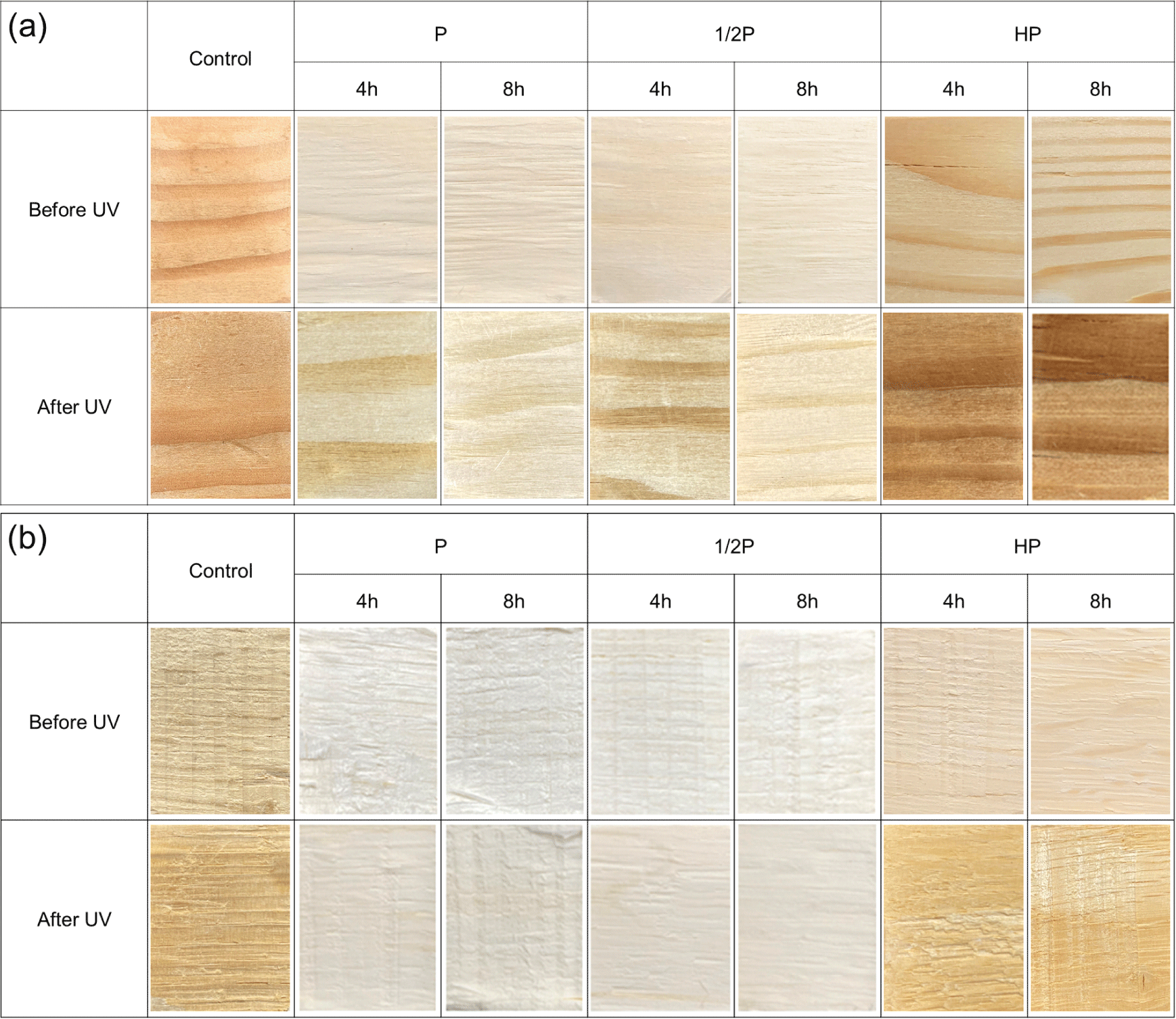
Visual analysis results revealed that the colors of both the tree species tended to lighten compared with control (untreated) when the PAA and HP treatments were performed. Compared to that of HP, more lightening was caused by the PAA treatment, and it was observed that the surfaces of the specimens lightened significantly with increasing solution concentrations and reaction times. Subsequently, the samples were exposed to UV light for 168 h in an accelerated weathering tester; the specimens treated with HP had a dark red color, but the specimens treated with PAA did not show a significant color change, demonstrating a wood photo-stabilization effect.
Meanwhile, surface fibers of the specimens treated with PAA showed tearing, indicating that the wood tissue softened considerably due to the delignification (Abdel-Fattah and Abdel-Naby, 2012; Kang et al., 2015; Westin et al., 2021).
In case of the hardwood Q. mongolica, the color was close to white after the PAA treatment better than HP treatment, and it experienced less color change after UV exposure compared to the softwood L. kaempferi. The color of Q. mongolica treated with PAA was similar at 4 h and 8 h. Therefore, 4 h of PAA treatment resulted in sufficient color stabilization. In the case of the L. kaempferi, the specimens treated by PAA turned yellow, and those treated by HP turned dark red. These observations are in accordance with the findings of several other studies that have reported that softwood tree species experience more severe color changes than hardwood tree species (Oberhofnerová et al., 2017; Timar et al., 2016).
Surface treatments were performed with varying treatment durations and concentrations of PAA and HP, and changes in wood color caused by the solutions were observed. Thereafter, the samples were exposed to UV light for 168 h, and the wood colors were observed. The lightness (L*), redness (a*), yellowness (b*), and color difference (ΔE*) values of the L. kaempferi and the Q. mongolica are shown in Fig. 4 and Fig. 5, respectively.
Both softwood and hardwood specimens exhibited similar tendencies for the same solution concentration and treatment duration except yellowness. In specimens treated with PAA, L* increased considerably and a* and b* decreased. In specimens treated with HP, L* and b* increased slightly compared to that in case of PAA and a* decreased.
After UV exposure, the L. kaempferi and the Q. mongolica demonstrated decrease in L* and increase in a*, but changes in b* differed for both species. For the L. kaempferi, b* increased after PAA treatment, and it remained the same or decreased after HP treatment. For the Q. mongolica, b* decreased after PAA treatment and increased after HP treatment. Because of this, when the color difference (ΔE*) was measured, the brown-colored lignin was found to have a relationship with the b* value, i.e., yellowness, and it tended to decrease with increasing solution concentration and treatment duration. b* of the PAA-treated specimens decreased rapidly compared to the untreated wood. b* decreases because chromophores are destroyed due to structural degradation during delignification (Pan et al., 2000). In contrast, compared to the untreated specimens, b* increased after HP treatment. This may be because of the structural changes in lignin, i.e., the conversion of benzene rings to quinones, after the HP treatment, which causes an increased reaction to UV light (Chen and Pignatello, 1997; More et al., 2021; Scheck and Frimmel, 1995).
For the PAA-treated specimens, the wood color did not change considerably. Similar to the surface treatment-induced color changes, the UV exposure-induced color changes were smaller in the PAA-treated specimens than in the HP-treated specimens. The color differences (ΔE*) of PAA-treated wood showed the 4.8–12.2 of L. kaempferi, and 1.7–3.7 of Q. mongolica, respectively. According to Table 2, under the condition of P-8, L. kaempferi is 9.0, which corresponds to large changes, and Q. mongolica is 2.4, which corresponds to small changes. Fig. 3(b) shwos that the E value of Q. mongolica under the condition P-4 is smaller than the value of P-8. This means that the lignin of Q. monglica, hardwood, is easily decomposed and has effective color stabilization even in a short time. Therefore, better wood photo-stabilization was achieved in the Q. mongolica sample than in the L. kaempferi sample.
This study used PAA, a strong oxidizing agent, for wood color stabilization. A similar study by Park et al. (2018a), Park et al. (2018b) reported photo-stabilization of wood using NaClO as an alkaline oxidizing agent. Chemical treatments were performed on birch and walnut veneers at 1%, 2%, and 3% concentrations, and the results showed that after exposing the veneers to UV light, the value of ΔE* was 5.4 for birch and 4.3 for walnut.
Another study by Yamamoto et al. (2017) analyzed color differences (ΔE*) under acid treatments using distilled water, HP, and alkali mixed in HP. Using a combination of alkali and HP, the ΔE* was 10, and it was 7 using a combination of HP and distilled water. Alkali or alkali mixtures have been typically used in wood color control studies; however, this study used PAA solutions so that the data can be used to compare alkali conditions and acid conditions (Hermansyah et al., 2019).
Fig. 6 shows the functional group changes in the wood surfaces of the untreated sample and the PAA and HP treatment, followed by UV exposure. The analysis was focused in the range of 2,000–800 cm–1 to observe changes in the lignin structure caused by the PAA and HP treatments. The functional groups assignments are listed in Table 3. Even though the peak positions are slightly different for the two wood species, the overall shapes of the peaks are similar. The notable difference between the softwood and hardwood is the C = O stretching band at 1,260 cm–1, which was only observed in the guaiacyl lignin of the L. kaempferi.
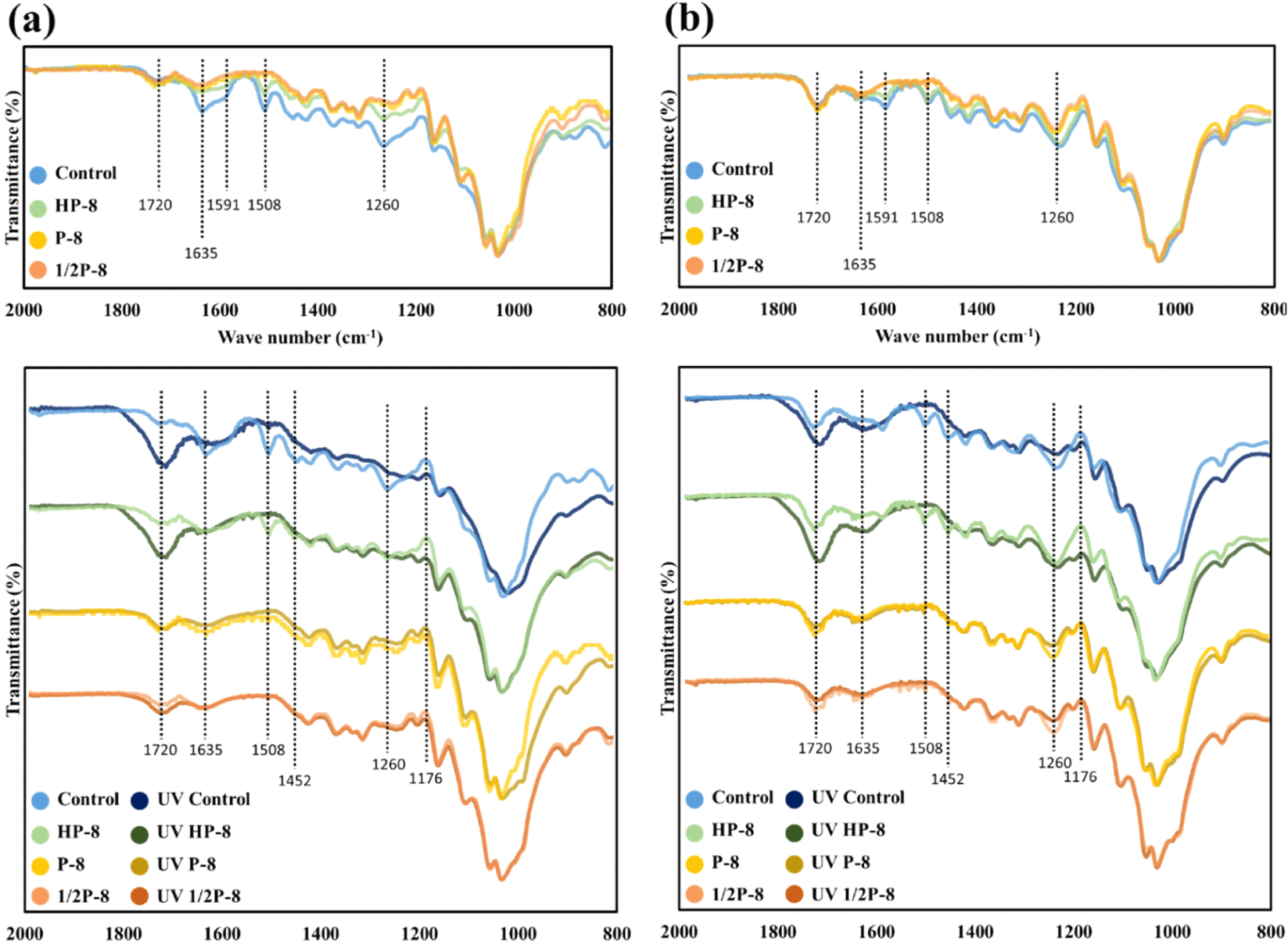
Reference: Schwanninger et al. (2004), Kwon et al. (2013), Miranda et al. (2012), Zhao et al. (2013), Xing and Li (2014), Timar et al. (2016), Abdullah et al. (2017), Oberhofnerová et al. (2017), Vaughn et al. (2017), Gonultas and Candan (2018), Yin et al. (2018), Zhang et al. (2018), Liu et al. (2016, 2019), Kubovský et al. (2020), Xing et al. (2020).
The top of Fig. 7(a) and (b) is a graph of the changes in the peaks of the untreated wood induced by the PAA and the HP treatments. The bottom of Fig. 6(a) and (b) is a graph of the changes caused by exposing the chemically surface-treated specimens to UV light. The cellulose C-O peak at 1,020–1,030 cm–1 indicates strong absorption and is not greatly affected, as revealed by FT-IR (Rosu et al., 2010). Therefore, similar to previous studies, intermittent quantitative analysis was performed by fixing the graph at 1,030 cm–1 and the changed peaks were observed. During the PAA treatment and artificial UV exposure, considerable changes occurred in the lignin-related functional groups, and small changes were found in the hemicellulose functional groups, indicating that some of the lignin and hemicellulose were partially removed. Specimens treated with PAA by irradiating UV light had no significant change in the aforementioned visual observation and color difference measurement. Also, in the FT-IR analysis, the PAA-treated specimen showed little change by UV light, so it was thought that PAA and UV light reacted with the same functional group. This is the reason for the wood stabilization reaction that occurs as the lignin that responds to UV irradiation is removed.
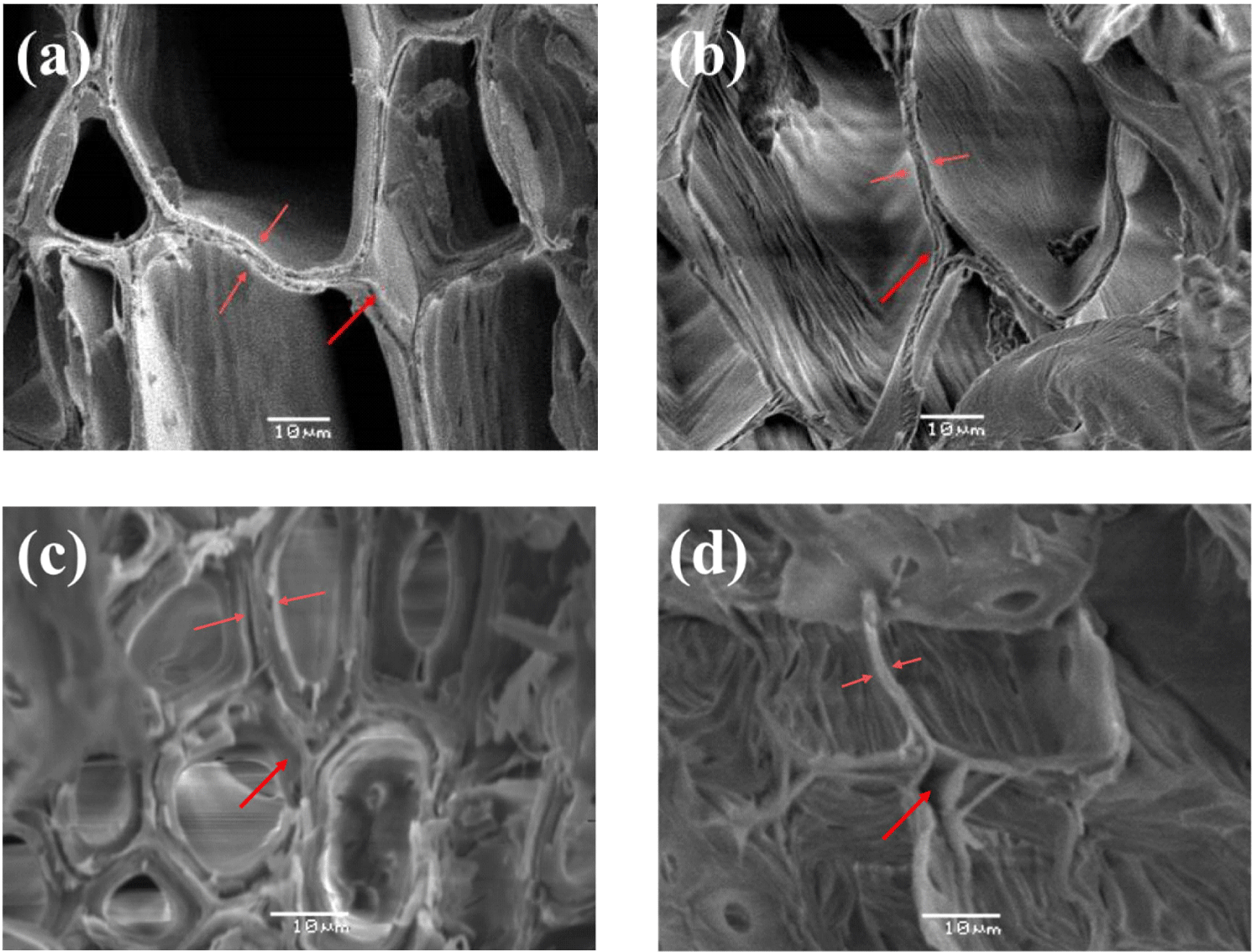
Fig. 7 shows scanning electron microscopy (SEM) images before and after PAA treatment. The cell walls in untreated samples were shown in the Fig. 7(a) and (c). After PAA treatment, empty spaces were observed between the cells [Fig. 7(b) and (d)]. These are the middle lamella that have high lignin content, and it was visually confirmed that the lignin in the cell walls was removed by the PAA.
Recent delignification studies have focused on the use of partially or completely delignified woods, which has expanded pores within the wood cells. And then, polymer impregnation or functional group modification is easier, resulting in new functional materials. Therefore, the proposed PAA delignification has many potential applications in manipulating cell walls and forming new materials.
Table 4 shows the extent of delignification via the measurement of depths from the surfaces of the two wood species after the chemical treatments. In the case of the PAA-treated specimens, removal of the thickest layer of lignin generally occurred along the direction of the fibers, and the penetration depth was proportional to the increase in concentration of PAA and the reaction time. This was attributed to the vessels and tracheid cells arranged along the direction of the fibers.
In the HP-treated specimens, which were the comparison group, the delignified areas were not distinct, and so the measurement was not conducted.
When the penetration depths of the two tree species were compared visually (Fig. 8), the delignification depth of L. kaempferi was deeper than Q. mongolica; however, a clear difference in the earlywood/latewood intermediate part was observed. In contrast, in the case of the Q. mongolica, there is a difference in the tissue cells including vessel elements because it is a hardwood species, and relatively uniform delignification was observed in the cross sections. Therefore, PAA treatment conditions should be set according to the wood species.
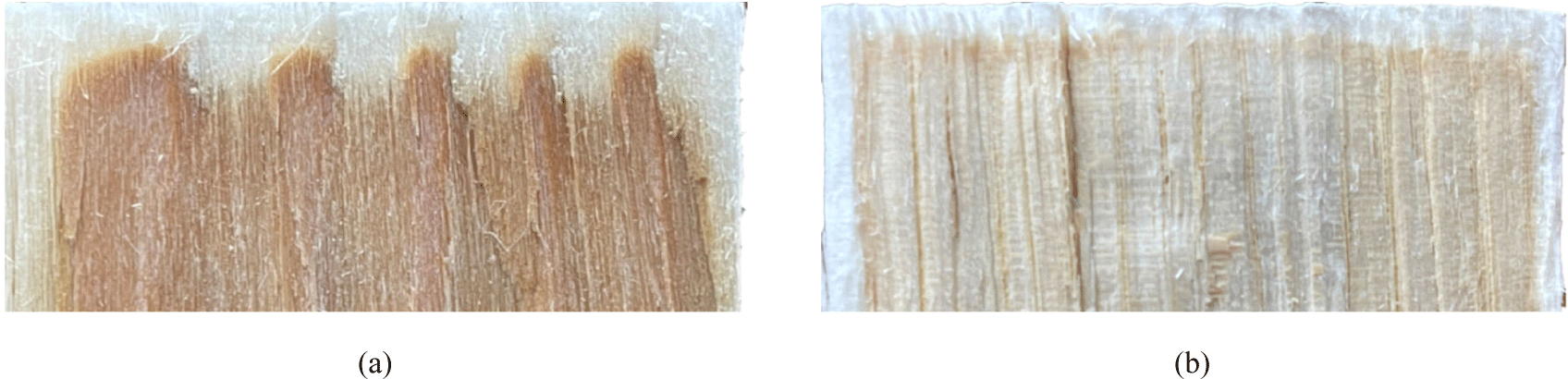
4. CONCLUSIONS
PAA and HP were used to perform surface chemical treatments on L. kaempferi and Q. mongolica specimens, and the treated samples were subsequently exposed to UV light. The following conclusions were obtained from observing the results of the treatments.
-
Visual observations indicated that the colors of both tree species tended to lighten after the surface chemical treatments. After subsequent UV exposure for 168 h, the color difference (ΔE*) in the PAA-treated L. kaempferi was 4.8–12.2, while the color difference in the Q. mongolica was 1.7–3.7. These results indicated that PAA treatment gives photo-stabilization effect of wood without significant changes.
-
FT-IR measurements indicated that there were major changes in the lignin-related functional groups as well as minor changes in the hemicellulose functional groups of the specimens treated with PAA. Thus, it can be inferred that some of the lignin and hemicellulose were removed by the surface chemical treatment.
-
SEM images indicated that lignin in middle lamella was removed and the cell walls became thinner, confirming that lignin can be selectively removed by PAA.
-
We determined that PAA can be effectively used for wood photo-stabilization, and it is an important method to control the degree of delignification by adjusting the time and the amount of PAA. Notably, surface tearing occurs during PAA treatment, and various methods such as densification and polymer impregnation can be used to prevent it.
In the future, it is essential to conduct studies on reducing the cost of the PAA treatment process by exploring ways to improve it and on the finding value-added chemical.