1. INTRODUCTION
In the background of the global problems regarding the use of fossil fuels, searching for renewable and clean energy has become a key issue. Undoubtedly, ethanol fuel is an optimum energy alternative for fossil fuels. This has fostered the development of renewable energy sources that are suitable for long-term use (Zabed et al., 2017; Hahn-Hägerdal et al., 2006). In this context, new strategies promoting the use of biomass for the future supply of energy, chemicals, and other useful materials are being studied (Van Dam et al., 2004; Jørgensen et al., 2007).
Lignocellulosic biomass such as woody biomass is regarded as a promising energy source because it is renewable and abundant in carbohydrates (Jung et al., 2017; Jo et al., 2016). Lignocellulosic biomass serves as a cheap and abundant feedstock, which is required to produce fuel ethanol from renewable resources at reasonable costs. Extensive research regarding the conversion of lignocellulosic biomass to ethanol has been performed in the last two decades (Sindhu et al., 2016; Maurya et al., 2015; Wright, 1998; Bjerre et al., 1996; Duff and Murray, 1996; Reshamwala et al., 1995; Cadoche and Lopez, 1989; Um et al., 2016).
From a long-term perspective, woody crops cultivated in short-rotation coppices, such as poplars and members of the genus Salix, are considered possible alternatives to the current sources of lignocellulosic biomass. Poplars, which are the most widespread species across Europe, represent an important woody crop cultivated in short-rotation coppices as a source of lignocellulosic biomass. In Sweden, different species of Salix have been the main option with regards to the utilization of short-rotation forests for the production of bioenergy, i.e. for the production of heat and power. Over the years, more frost-tolerant and pest-resistant Salix varieties with considerably higher productivities have been developed through breeding, selection, and plantation management (Bawelin, 2002; Larsson, 1999; Tharakan et al., 2003). Instead of only producing heat and power by burning the Salix chips as they are, it has been proposed that the carbohydrate fraction (cellulose and hemicellulose) could be used for the production of fuel ethanol, and the solid residue, which consists mainly of lignin, could be used as a solid fuel. A dedicated woody biomass feedstock production system based on the short-rotation intensive culture (SRIC) of fast-growing willow clones is being developed for the northeastern and mid-western United States (Abrahamson et al., 1998). Populus species, along with species from its sister genus Salix, will provide valuable feedstock resources for advanced second-generation biofuels. Their inherent fast growth characteristics can particularly be exploited for managing short rotation, which is a time- and energy-saving alternative cultivation method for producing lignocellulosic feedstock (Porth and El·Kassaby, 2015).
The short-rotation coppice is a system to maximize the unit production of biomass by reducing the growth period of the crops. The most important short-rotation woody crops cultivated in the Republic of Korea are the hybrids Populus nigra × Populus maxiwiczii, Populus euramericana, Populus alba × Populus glandulosa, and Salix alba. Most of the biomass produced from these crops are used as pellets or chips, and only a limited amount of biomass is used as a source for producing bioethanol (Kim et al., 2011; Kim et al., 2015).
Therefore, in the present study, we evaluated the potential of branches from immature (3-year-old) and mature (12-year-old) short-rotation woody crops to serve as biomass resources for bioethanol production. We then analyzed the chemical compositions and compared the glucose conversion by performing the enzymatic hydrolysis of non-pretreated short-rotation woody crop branch samples. Finally, we investigated the effects of the growth period of the crops on the ethanol production by fermenting the converted enzymatic hydrolysates.
2. MATERIALS and METHODS
Woody crops cultivated in short-rotation coppices (Populus nigra × Populus maxiwiczii, Populus euramericana, Populus alba × Populus glandulosa and Salix alba) were collected from an experimental plot at the Korea Forest Research Institute in the city of Seoul, Republic of Korea (Fig. 1). Branches from 3-year-old and 12-year-old crops were debarked, chipped, and ground with a Wiley mill, and the fraction between 20-mesh screen and 80-mesh screen was collected for further use. This material was then stored at room temperature.
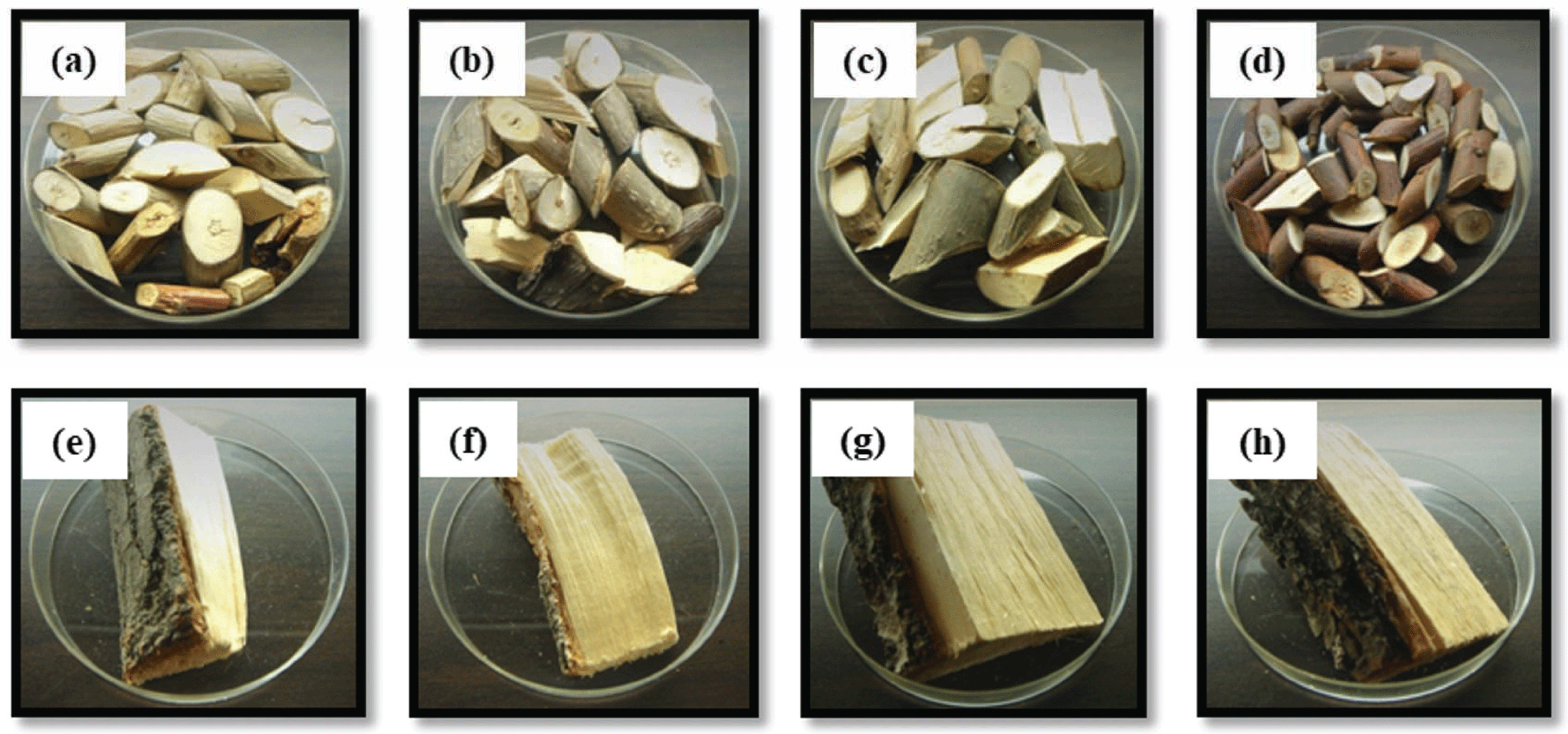
The raw material composition was determined according to the analytical methods for biomass described by the National Renewable Energy Laboratory (NREL, Golden, CO). The extractive content was determined based on the solubilized material obtained after a Soxhlet extraction with ethanol for 24 h. The ash content was determined according to the method involving the calcination of raw materials at 550°C. The cellulose and hemicellulose contents were determined based on the monomer content measured after a two-step acid hydrolysis procedure to fractionate the fiber. The first step was performed by treating the samples with 72% H2SO4 at 30°C for 60 min. In the second step, the reaction mixture was diluted until the H2SO4 concentration reached 4%, and then autoclaved at 121°C for 1 h. This hydrolysate was then analyzed for sugar content by high-performance liquid chromatography (HPLC) using a Waters 2695 liquid chromatography system with a refractive index detector. An Aminex HPX-87P carbohydrate analysis column (Bio-Rad, Hercules, CA) operating at 85°C with deionized water as the mobile-phase (0.6 mL/min) was used. The solid residue remaining after this acid hydrolysis process was considered the acid-insoluble lignin portion. The acid-soluble lignin in the hydrolysates was also quantified. The acid-soluble lignin content was determined based on UV spectrophotometric measurements at 205 nm.
The enzymes used for the enzymatic hydrolysis were Celluclast 1.5® L (Novo Co., Denmark), which is cellulase prepared by a culture solution of Trichoderma reesei, and Viscozyme® L (Novo Co., Denmark), a β-glucosidase. One gram of biomass was transferred to a 250-mL Erlenmeyer flask, followed by the addition of 50 mL of 0.1 M citrate buffer (pH 4.8). Next, appropriate amounts of cellulase (65 FPU/g) and β-glucosidase (24 CBU/g) were added. The flask was placed in a shaking incubator (IS-97IR from Jeio-Tech Co., Korea) at 50°C and 150 rpm and incubated for 96 h. Samples were withdrawn after 0, 12, 24, 48, 72, and 96 h to monitor the progress of hydrolysis. Each sample taken from the hydrolysis solution was heated to 100°C immediately for 10 min to denature the enzymes, cooled to room temperature, and then centrifuged for 15 min at 3,000 rpm (Hanilmicro-12 from Hanil Science Industrial Co., Korea). The supernatant was used for glucose analysis using an HPLC system (Shimadzu, Kyoto, Japan) with a refractive-index detector (Shimadzu), and the sample containing glucose was utilized for yeast fermentation.
The percent glucose conversion was calculated as follows (Eq. (1)):
where GH is the dry-weight percentage of glucose in enzyme hydrolysis supernatant (g glucose/g solids hydrolyzed), and GP is the dry-weight percentage of glucose in the raw material (g glucose/g the raw material).
Fermentation of the liquids was performed using the ordinary yeast strain Saccharomyces cerevisiae KCTC 7296, which ferments glucose and mannose, but not xylose or other pentoses. The inoculum was prepared by transferring the organisms cultured on GPYA medium (glucose, 40 g·L-1 peptone, 5 g·L-1 yeast extract, 5 g·L-1 agar, 15 g·L-1) into a 100-mL flask. The culturing was carried out at 35°Con an orbital shaker for 24 h. The inoculum concentration was about 1.5 × 108 yeast cells/mL. The pH was adjusted to 5.5 with 20% (w/w) Ca(OH)2. The fermentation was performed in 100-mL glass flasks with a working volume of 20 mL; the fermentation solution consisted of 18.5 mL of filtrate, 0.5 mL of nutrients, and 1 mL of inoculum (an aqueous suspension of yeast). Yeast was used at a concentration of 1.5 × 108 yeast cells/mL, while the final concentrations of the nutrients were 0.5 g·L-1 (NH4)2HPO4, 0.025 g·L-1 MgSO4·7H2O, 0.1 M NaH2PO4, and 1 g·L-1 yeast extract (Taherzadeh et al., 1996). The flasks were sealed with rubber stoppers through which hypodermic needles had been inserted for the removal of the CO2 produced, as well as for the withdrawal of samples. The concentration of fermentable sugars was adjusted by the addition of glucose to a total concentration of 5 g·L-1 to avoid the influence of the variation in sugar concentration between different filtrates. A reference solution was prepared with 5 g·L-1 glucose to serve as a control fermentation sample. The flasks were incubated at 35°C for 96 h, and the withdrawn samples were analyzed for sugars and ethanol. Samples were withdrawn after 0, 12, 24, 48, 72, and 96 h to monitor the progress of the fermentation process.
The content of monomeric sugars in the enzymatic hydrolysates (glucose) was analyzed using an HPLC system (Shimadzu, Kyoto, Japan) with a refractive-index detector (Shimadzu). The separation of the sugars was performed using an Aminex HPX-87P column (Bio-Rad, Hercules, CA, USA) at 85°C, with water as the eluent, and at a flow rate of 0.6 mL/min.
Additionally, the ethanol contents of the fermentation samples were analyzed using an Aminex HPX-87 H column (Bio-Rad) at 65°C, with 5 mM H2SO4 as the eluent, and ata flow rate of 0.6 mL/min (Sluiter and Hames, 2004).
All samples were filtered through a 0.2 μm filter before analysis to remove particulate matter. All analytical determinations were performed in duplicate and the average results are shown.
3. RESULTS and DISCUSSION
Woody crops have high contents of cellulose and hemicellulose, which can be readily hydrolyzed into fermentable sugars (Binod et al., 2010). Cellulose (as glucose) can either be broken down hydrolytically or enzymatically (by cellulases) into glucose. Glucose, galactose, and mannose, which are six-carbon sugars (hexoses), are readily fermented to ethanol by many naturally occurring organisms, but the pentoses xylose and arabinose (containing only five carbon atoms) are fermented to ethanol only by a few native strains, and usually at relatively low yields (Mosier et al., 2005).
Table 1 summarizes the chemical composition of 3-year-old and 12-year-old P. nigra × P. maxiwiczii, P. euramericana, P. alba × P. glandulosa, and S. alba branches. The cellulose portion of the 3-year-old P. nigra × P. maxiwiczii, P. euramericana, P. alba × P. glandulosa, and S. alba branches comprised 44.2%, 46.0%, 48.6%, and 44.1% glucose, respectively. These branches also comprised 18.1%, 18.9%, 19.9% and 18.0% of hemicellulosic sugars as the main sugar, and xylose (14.0–15.5%), galactose (1.0–2.2%), arabinose (1.0–2.0%), and mannose (1.0–1.1%) were the other additional available carbohydrates (data not shown). The lignin content from these branches (P. nigra × P. maxiwiczii, P. euramericana, P. alba × P. glandulosa, and S. alba) ranged from 21.4–26.8%, the extractive content ranged from 6.5–9.6%, and the ash content ranged from 1.1–2.0%.
In the 12-year-old P. nigra × P. maxiwiczii, P. euramericana, P. alba × P. glandulosa, and S. alba branches, the cellulose content ranged from 45.4–47.3%, the hemicellulose content ranged from 18.6–20.2%, the lignin content ranged from 23.5–27.8%, the extractive content ranged from 4.6 to 7.0%, and the ash content ranged from 1.2–1.7%.
The carbohydrate content (cellulose and hemicellulose) of woody crops cultivated in short-rotation coppices has been reported to be higher than that in case of other woody plants and agricultural residues (Jorgensen et al., 2007; Zhu and Pan, 2010). We expected that longer harvest ages will produce increased contents of carbohydrate and lignin. However, the carbohydrate and lignin contents of the 3-year-old and 12-year-old woody crop branches showed no significant difference. Therefore, the immature wood samples, i.e. branches from woody crops grown in short-rotation coppices for a short period may be promising options as sources of feedstock for bioethanol production, especially in terms of their abundant availability and low costs.
Enzymatic hydrolysis of the non-pretreated raw materials from the 3-year-old and 12-year-old woody crop branches was carried out. The glucose conversion of the 3-year-old and 12-year-old woody crop branch samples has been presented in Fig. 2. The glucose conversion in the 3-year-old P. nigra × P. maxiwiczii, P. euramericana, P. alba × P. glandulosa, and S. alba branches was 40%, 26%, 29%, and 26%, respectively; the glucose conversion in the 12-year-old P. nigra × P. maxiwiczii, P. euramericana, P. alba × P. glandulosa, and S. alba branches was 24%, 21%, 22%, and 19%, respectively. The maximum glucose conversion was attained in case of the 3-year-old P. nigra × P. maxiwiczii branches (40%), and the lowest glucose conversion, in case of the 12-year-old S. alba branches. Thus, it was seen that the glucose conversion in the 3-year old woody crop branch samples (26–40%) was higher than that in the 12-year-old woody crop branch samples (19–24%). Significant difference between the chemical compositions of the 3-year-old and 12-year-old woody crop branch samples was not observed (Table 1), but different results were obtained with regards to glucose conversion.
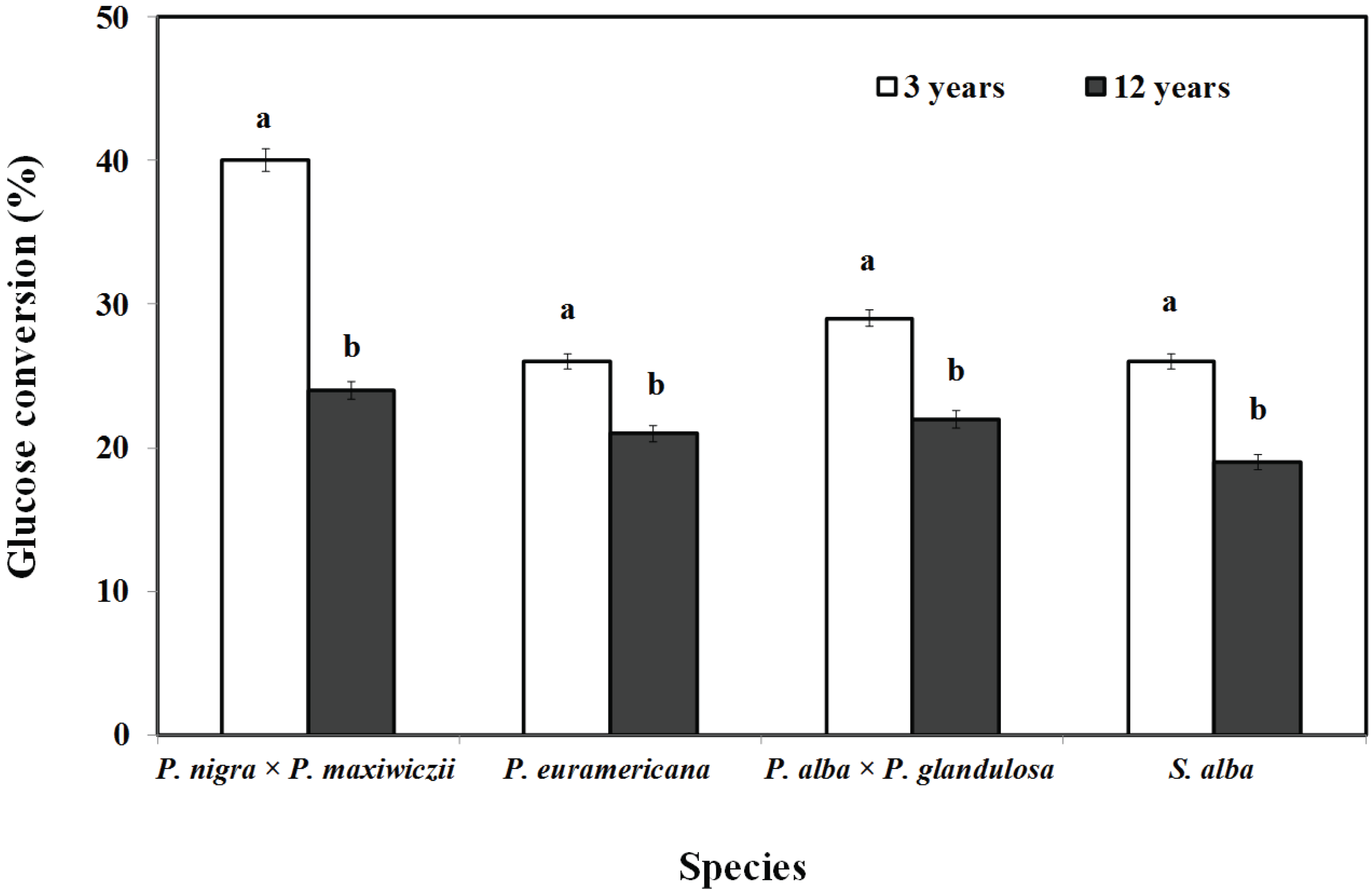
We estimate that this difference is because of physical characteristics such as crystallinity, hardness, or specific gravity due to growth periods. Wood lignification creates a physical barrier for enzymatic attack on the polysaccharides during the growth periods. Therefore, only those organisms that possess enzymes capable of destroying the lignin or at least altering its protective association with the polysaccharides, are capable of decaying wood. The structure of cellulose, which comprises crystalline and amorphous regions, also restricts the action of depolymerizing enzymes. Initially, these enzymes can only affect the non-crystalline portions (Quartey, 2009).
Batch fermentation was carried out with media comprising hydrolysates of the branches from woody crops cultivated in short-rotation coppices (P. nigra × P. maxiwiczii, P. euramericana, P. alba × P. glandulosa, S. alba) to investigate the time profiles of sugar consumption and ethanol formation. The time courses of the fermentation processes are shown in Fig. 3 – Fig. 6.
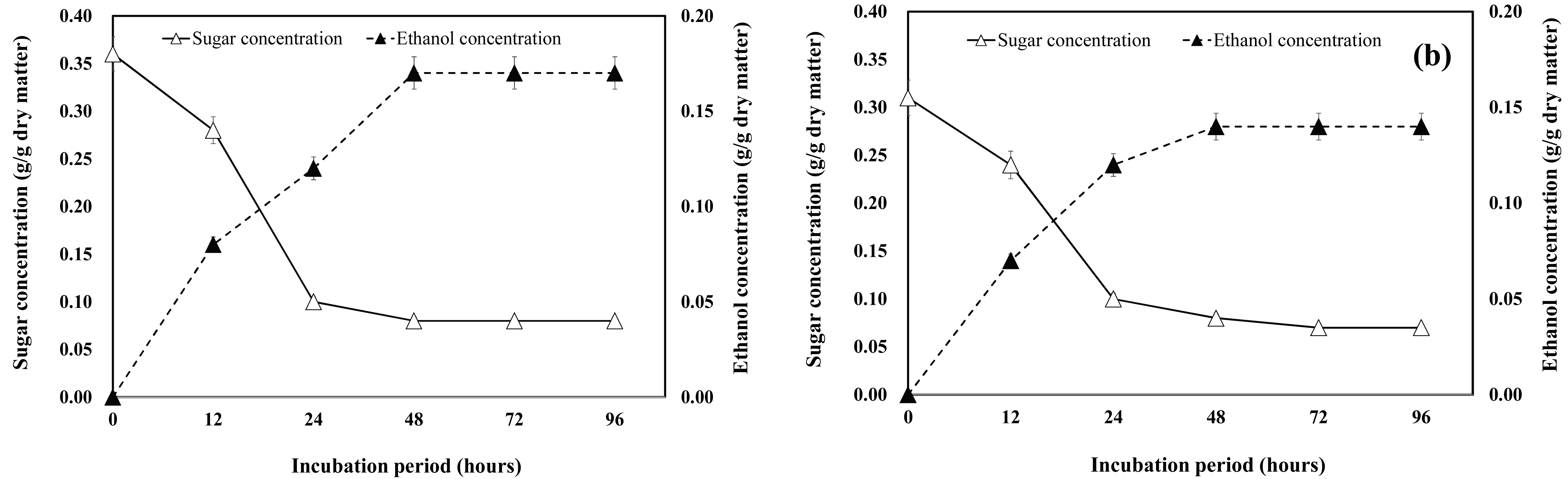
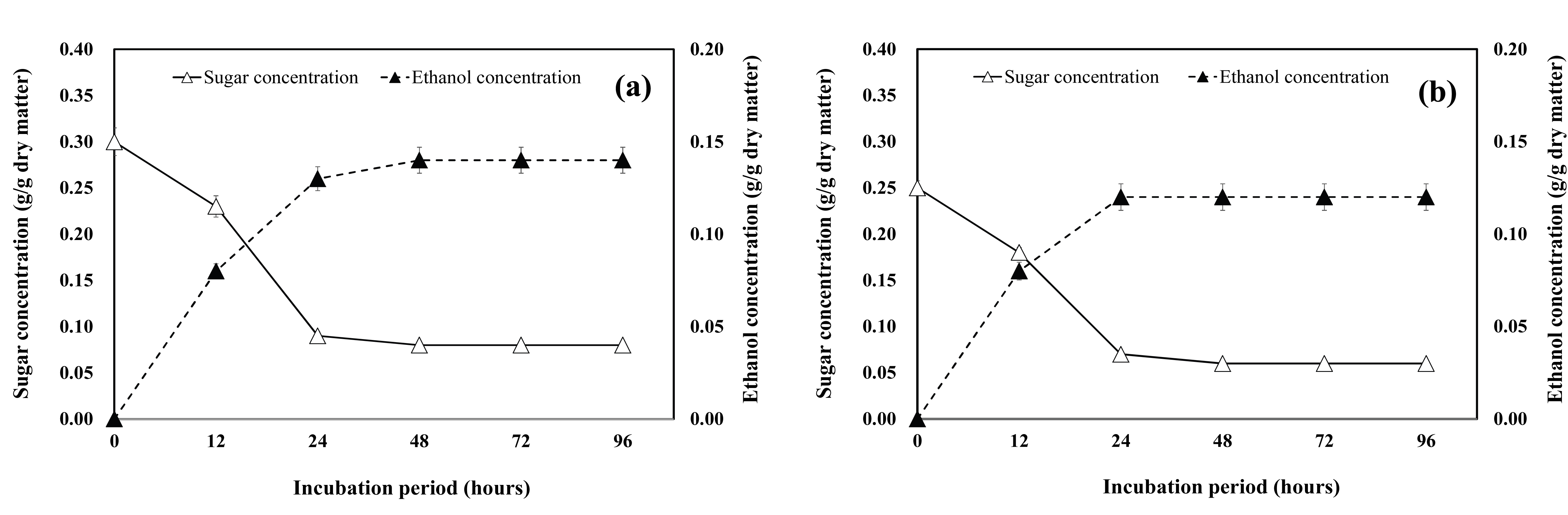
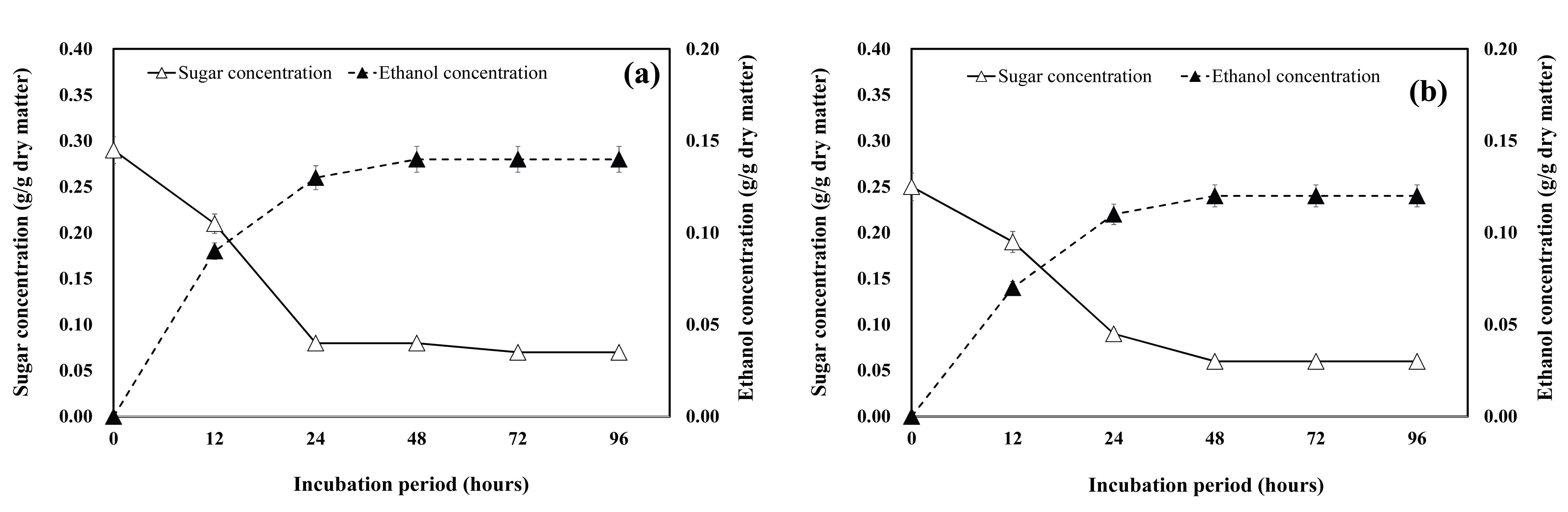
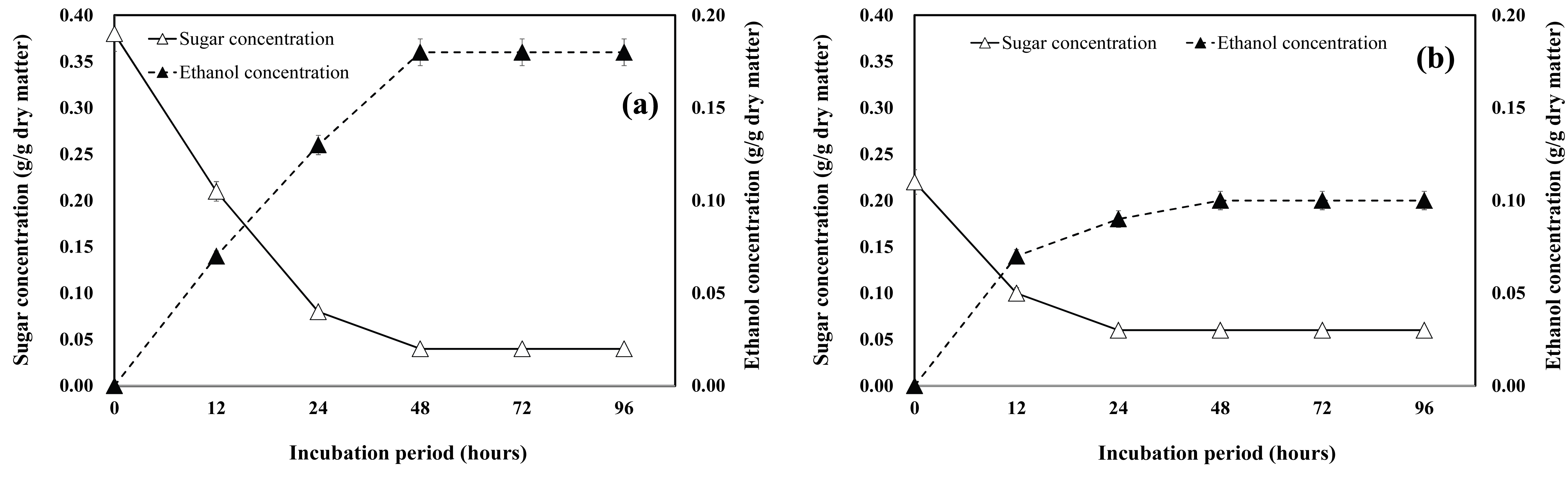
The sugar consumption and ethanol production from P. nigra × P. maxiwiczii branches is shown in Fig. 3. The time course of ethanol production showed a similar pattern for the hydrolysates of both the 3-year-old and 12-year-old P. nigra × P. maxiwiczii branch samples (Fig. 3a and Fig. 3b). At the time point (48 h) when the maximum concentration of ethanol was attained, the ethanol conversion rate was over 90%. The ethanol concentrations obtained from the fermentation of the 3-year-old and 12-year-old woody crop branch hydrolysates were 0.17 g/g dry matter and 0.14 g/g dry matter, respectively.
As shown in Fig. 4a and Fig. 4b, the ethanol concentrations of the hydrolysates of both the 3-year-old and 12-year-old P. euramericana branch samples increased significantly with the increase in sugar consumption. The ethanol conversion rate was over 93%, and the maximum ethanol concentration (0.14 g/g dry matter) was obtained in case of the hydrolysates of the 3-year-old woody crop branches.
The sugar consumption and ethanol production in the P. alba × P. glandulosa branch samples is shown in Fig. 5. The ethanol conversion in case of the substrates derived from the 3-year-old or 12-year-old P. alba × P. glandulosa branch samples (Fig. 5a and Fig. 5b) was similar, reaching 96.6% and 96.0%, respectively. Furthermore, the maximum ethanol concentration (0.14 g/g dry matter) was obtained after incubating the hydrolysates of the 3-year-old woody crop branch samples for 48 h.
The time course of sugar consumption and ethanol production using the enzymatic hydrolysates from the 3-year-old and 12-year-old S. alba branches is shown in Fig. 6a and Fig. 6b. The highest concentration of ethanol reached 0.18 g/g dry matter and 0.10 g/g dry matter after 48 h of fermentation in case of the 3-year-old and 12-year-old S. alba branches, respectively.
The correlation between ethanol production and reducing sugar content was high; the ethanol production inversely correlated with sugar consumption. Further, the sugar concentration decreased during the fermentation, coinciding with an increase in ethanol production. The sugar concentration fell rapidly and consistently during the first 24 h of fermentation, after which it decreased slowly. When the enzymatic hydrolysates of the short-rotation woody crops were fermented, the ethanol conversion rate was over 90%, and as expected, the ethanol concentration was higher after the fermentation of the 3-year-old woody crop branch sample hydrolysates, than after the fermentation of the 12-year-old woody crop branch sample hydrolysates.
4. CONCLUSION
We investigated the potential of branches from immature (3-year-old) and mature (12-year-old) short-rotation woody crops (Populus nigra × Populus maxiwiczii, Populus euramericana, Populus alba × Populus glandulosa, and Salix alba) to serve as feedstock for bioethanol production. This study showed that there was no significant difference between the cellulose, hemicellulose, and lignin contents of the branches from 3-year-old and 12-year-old woody crops cultivated in short-rotation coppices. However, after the enzymatic hydrolysis, the glucose conversion was found to be higher in the branches from the 3-year-old short-rotation woody crops (glucose conversion: 26–40%)) than in branches from their 12-year-old counterparts (glucose conversion: 19–24%). Likewise, after fermentation, the ethanol concentration was found to be higher in the branches from the 3-year-old short-rotation woody crops (~ 0.18 g/g dry matter)) than in branches from their 12-year-old counterparts (~ 0.14 g/g dry matter).
Our results showed that the immature (3-year-old) wood samples (from crops grown for short periods) can be used as a biomass source for producing bioethanol. We recommend that further studies on aspects such as the biomass contents produced per unit area and after the removal of bark from the branches be carried out.