1. INTRODUCTION
Kapok [Ceiba pentandra (L.) Gaertn] is a fast-growing wood with low physical and mechanical properties as well as natural durability. Its density ranged from 0.23–0.37 g/cm3 with an average of 0.30 g/cm3 (Amin and Dwianto, 2006; Brown, 1997), but the potential is high due to being widely planted as a fiber producer. Considering this low density, kapok wood is only used for non-structural materials such as cast boards. Since there is a shortage of supply from natural forests, fast-growing species have become a raw material source for the wood industry, originating from the plantation and rural forests. The wood from plantation forests is commonly fast-growing species with low physical and mechanical properties. The low density and dimensional stability are the major weaknesses in these physical properties (Ghani and Lee, 2021; Priadi et al., 2019). Therefore, wood modification is developed with the main objective of improving the physical and mechanical properties (Ghani and Lee, 2021). Densification can augment the usefulness of species with low density in order to improve their physical and mechanical properties (Fang et al., 2012; Laine et al., 2016; Navi and Girardet, 2000). This process increases the density, strength, hardness, and resistance of wood to microorganisms (Esteves et al., 2017; Gašparik and Gaff, 2015; Schwarze and Spycher, 2005).
The main densification problem is reducing the set-recovery of densified wood (Dwianto et al., 1999; Neyses et al., 2017). Based on previous reports, the reduction in set-recovery has been carried out through heat treatment (Lee and Lee, 2018; Priadi et al., 2019), chemical modification (Hadi et al., 2022; Jain et al., 2022), or a combination of heat and steam (Laine et al., 2013) plus saturated steam (Kutnar and Kamke, 2012). Esteves and Pereira (2009) noted that heat/steam treatment before or after densification decreases set-recovery as an implication of increasing wood dimensional stability. This reduction can also be accomplished by combining densification with chemical modification such as acetic anhydride (Laine et al., 2016), furfuryl alcohol (Westin et al., 2009), styrene (Devi et al., 2003), acetic acid and hydrogen peroxide (Yunianti et al., 2019), boron (Augustina et al., 2020), and poly(vinyl)alcohol (Sun et al., 2016). However, several treatments have not been satisfactory for decreasing set-recovery due to not achieving fixation between chemical components during densification.
Densified wood set-recovery may occur due to stress relaxation after the compression process or the effect of moisture caused by temporary fixation. The fixation can be increased through hemicellulose degradation and partial depolymerization of lignin (Dwianto et al., 1999). Furthermore, the decrease in the amorphous fraction of the wood components possibly promotes dimensional stability due to reduced hygroscopicity. According to Fengel and Wegener (1984), the dimensional stability of wood is strongly influenced by wood hydroxyl groups, which are abundant in hemicellulose and amorphous cellulose. In alkali conditions, hemicellulose and cellulose as well as lignin can be initially degraded and dissolved in a mild (1%) NaOH solution (Fatrawana et al., 2019; Maulana et al., 2021; TAPPI, 1996). Therefore, alkali treatment before densification should increase dimensional stability or decrease set-recovery. Neyses et al. (2017) stated that the pretreatment with sodium silicate, sodium hydroxide, ionic liquid, and methacrylate increased dimensional stability. However, the set-recovery was not significantly decreased, probably due to chemical treatment applied only on the wood surface. Supposing chemical pretreatment is enough to remove the amorphous parts in wood components it may increase the dimensional stability. This research aims to improve the dimensional stability of densified wood by pretreatment with an alkali solution, and changes in chemical composition in the selected sample which may affect the increased dimensional stability were evaluated. Kapok wood, known as the light wood of fast-growing tropical species, was used in this study.
2. MATERIALS and METHODS
Kapok (C. pentandra) logs were obtained from Subang Regency, West Java, Indonesia. These were cut into specimens with dimensions of 35 cm × 5 cm × 5 cm. Samples for chemical composition analysis were prepared according to the TAPPI standard 257 om-85. The wood meals were made through grinding with a Willey mill followed by sieving to collect particles of 40–60 mesh sizes.
The alkali pretreatment procedure was referred to as the method carried out by Song et al. (2018) with modification. The wood samples were air-dried to 14%–16% moisture content (MC) and then boiled in 1.25 N NaOH solution for 1, 3, and 5 h. The treated samples were then washed with hot distilled water for 10 minutes twice. Afterward, they air-dried for two weeks before being compressed in the densification step, and the initial dimension of the wood specimens was measured. Alternatively, a controlled oven or kiln drying at low temperatures (40°C–60°C) may be considered to shorten drying time.
The 5 cm-thick samples were densified with a thickness-decreasing target of 80% from the initial dimensions. The compression was carried out in a tangential direction at a temperature and pressure of 100°C and 15 Mpa for 5 h, followed by a cold pressing for 10 h. Subsequently, the test samples were kept at room condition for one week.
The physical properties of wood including MC, density and specific gravity (SG) were determined from a small clear specimen. The test sample with a dimension of 1 cm × 1 cm × 1 cm size was weighed initially, then dried in an oven for 24 h at 105°C, cooled in a desiccator, and weighed again. The MC and SG were calculated with the following Equation (1).
MC: moisture content (%), W0: initial weight (g), W1: oven dried weight (g), SG: specific gravity, V: volume of wood, D: density of wood (g/cm3).
The dimensional stability was evaluated by ASE value, which was determined based on a comparison of the swelling coefficient of treated and untreated samples. The test samples were dried in an oven at a temperature of 103°C for 48 h, then immersed in water for 24 h. Their weight and volume were measured before and after immersion. The ASE was calculated with the following Equation (2).
ASE: anti-swelling efficiency (%), Su: swelling coefficient of untreated sample (%), St: swelling coefficient of the treated sample (%).
The chemical composition of densified, alkali-treated, as well as alkali-pretreated and densified woods were analyzed on the selected sample with the highest ASE value. This analysis evaluates the change in chemical composition that affected dimensional stability due to the treatment.
Holocellulose represents the content of cellulose and hemicellulose in wood. About 80 mL of distilled water, 1 g of sodium chlorite (NaClO2), and 0.5 mL of glacial acetic acid (CH3COOH) were added to a 2 g extractive-free wood meal, and heated in a water bath at 70°C–80°C. The addition of sodium chlorite and acetic acid was repeated after every one-hour reaction until four times. The sample was filtered using a glass filter and rinsed with hot distilled water, then oven-dried at 105°C for 24 h. The holocellulose content was expressed as a percentage of holocellulose residue to the sample dry weight.
The cellulose content was expressed by α-cellulose. It was determined from holocellulose by adding 10 mL of 17.5% NaOH solution at 20°C and stirring to facilitate contact between the wood and chemical. After five minutes, 5 mL of the 17.5% solution was added three times until the total NaOH volume became 25 mL. The reaction was kept for 30 minutes to obtain a total of 45 minutes. To the sample, 33 mL of distilled water was added and stirred, and the reaction was maintained for the next 1 h at 20°C. This was filtered using a glass filter, then rinsed with 100 mL of 8.3% NaOH and distilled water, after which it was dried at 105°C and weighed. The α-cellulose content was expressed as a percentage of the weight of residue in the dry sample. The laboratory grade of NaClO2 and NaOH (Wako Pure Chemical, Osaka, Japan), CH3COOH (Merck, Darmstadt, Germany), and other chemicals were used without further purification.
Lignin content was determined according to TAPPI T 222 om-88 with modifications (Dence, 1992). A 0.5 g extractive-free sample was hydrolyzed with 5 mL of 72% sulfuric acid (H2SO4, Merck Germany). The mixture was reacted in room condition for 3 hours, and the solution was diluted to get a concentration of 3% H2SO4. The reaction was continued at 121°C for 30 minutes with an autoclave. Lignin residue was precipitated, filtered, and rinsed with hot distilled water until acid-free, then dried at 105°C for 24 h. Its content was expressed in percentage to the dry sample weight.
The Fourier Transform Infra-Red (FTIR) analysis was used to indicate the variation of wood components by measuring functional groups change. The functional groups were characterized with the Attenuated Total Reflectance-Fourier Transform Infra-Red (ATR)-FTIR (Perkin Elmer, Shelton, CT, USA). Furthermore, 0.1 mg wood was analyzed, and the IR spectrum was recorded with ten scans per sample. Measurements were carried out in absorption mode and at a spectral resolution of 4.0 cm–1, in wave numbers 4,000 to 600 cm–1.
Samples were analyzed under X-ray Diffraction (XRD) chamber conditions recorded with the Shimadzu XRD-700 Maxima X series. NI radiation was filtered with CuKα at a wave number of 0.1542 nm. X-rays were operated at a voltage of 40 kV and 30 mA, as well as a scan angle 2°C by 10°–40° every 2° per minute. The materials’ crystallinity index (CI) was calculated according to Zhao et al. (2006) from the diffraction intensity data with the following formula:
Fc means a crystalline region, and Fa is the non- crystalline/amorphous region. The total intensity was calculated using the software contained in the Shimadzu MaximaX diffractometer.
3. RESULTS and DISCUSSION
Density change is the main parameter in densified wood products due to a reduction in dimensions. Kapok wood has a density of 0.25 g/cm3, which was increased by 3.56 times to 0.87 g/cm3 through densification. The initial alkali pretreatment elevated the density by 15% compared to densified wood, as indicated in Fig. 1. Conduction of this process for 1, 3, and 5 hours generated wood with similar densities. Densification for all samples was carried out with a target of reducing thickness to obtain relatively similar density. However, alkali treatment softens wood and densification produces a more compact structure with better dimensional stability. A mild alkali solution may degrade and dissolve components of wood cell walls with low molecular weights (TAPPI, 1996). The partial removal of hemicellulose and lignin in an alkali solution was reported from bamboo flakes by rinsing treatment (Fatrawana et al., 2019). The statistical analysis showed that the NaOH treatment had a significant effect on the density of compacted kapok, while the 1, 3, and 5 hours boiling time did not affect the results.
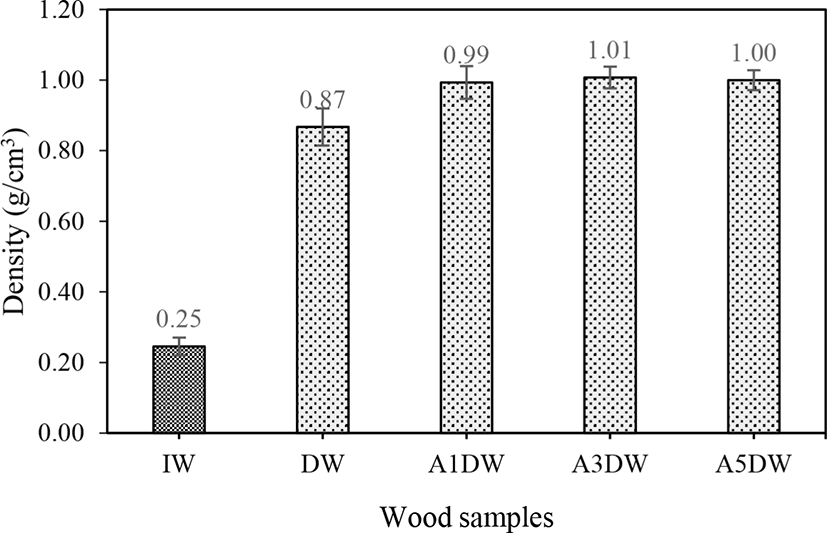
Alkali-pretreated and densified wood had lower MC than the densified wood, as shown in Fig. 2. Moreover, their hygroscopic properties were reduced by the compacted structure and heat applied during compression. Alkali pretreatment for 1 h of boiling decreased the MC, while it was increased by the 3 and 5 h time. Longer boiling time increased cell wall component degradation, and it is suggested to cause the elevation of hydroxyl groups. Wood absorbs and releases water due to being hygroscopic. A decrease in the absorption capacity is facilitated by the densification process plus heat. The NaOH solution degrades hemicellulose, hence, the amorphous fractions that play a role in water absorption and molecules binding diminish. According to Jamsa and Viitaniemi (2001), the decline in amorphous fractions could decrease cell wall hydrophilic properties through the reduction of hydroxyl groups. ANOVA showed the significant effect of treatments on the MC. Duncan’s further test indicated that alkaline-treated and densified wood significantly differed from the control sample.
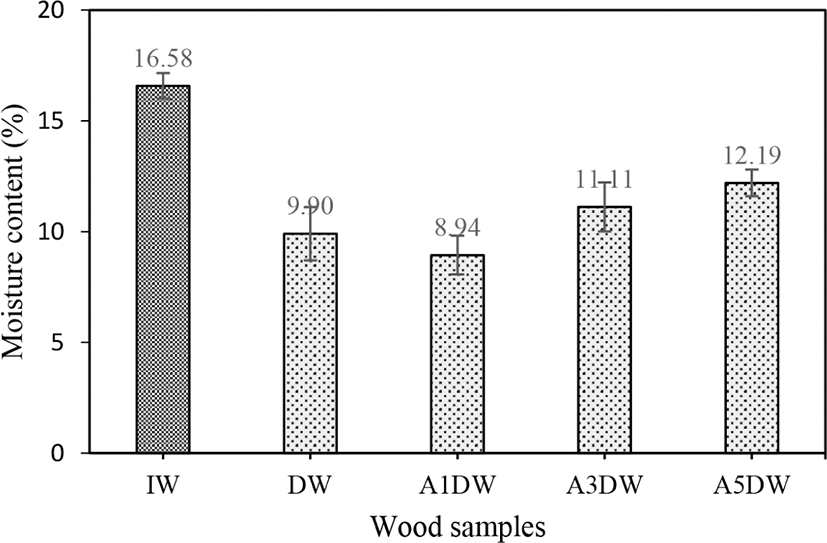
ASE is used as an indicator of the dimensional stability of wood before and after being treated with certain modifications, where a high ASE value signifies great dimensional stability. The ASE value obtained in this research can be seen in Fig. 3. The variance analysis results showed that the boiling in 1.25 N NaOH solution for 1, 3, and 5 h had a significant effect on densified kapok wood ASE. Based on Duncan’s test, 3 h of boiling time produced ASE values significantly different from 1 h but not significantly different from 5 h. Boiling in NaOH solution for 3 h increased the ASE of 6.4 times compared to 1 h boiling, but prolonging boiling time to 5 h slightly decreased the ASE value by 22.6%, and statistically not different. A study by Song et al. (2018) showed that 2.5 N NaOH and 0.4 N Na2SO3 pretreatment reduced densified wood swelling from 43.1% to 8.4%. The combined soaking of NaOH 10% for 1–3 h and densification increased dimensional stability by a lower compression set ratio (Augustina et al., 2022).
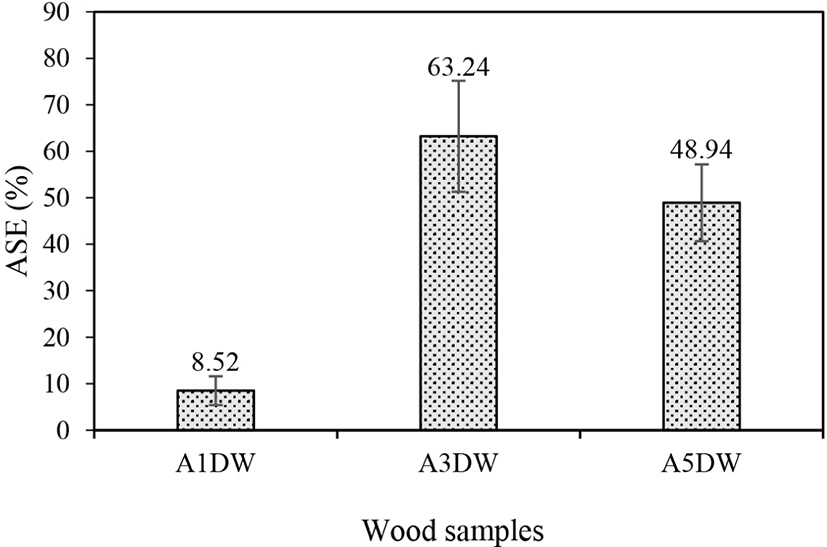
The pretreatment in 1.25 N NaOH solution elevated densified kapok wood ASE, with the highest value of 63.24% obtained by 3 h boiling. The longer alkali treatment at high temperatures is thought to promote higher wood components’ degradation. The alteration in the dimensional stability of alkali-pretreated and densified wood is thought to be initiated by changes in wood properties due to partial degradation or dissolution of chemical components. Alkali solution partially extracts lignin and carbohydrates, specifically hemicellulose and cellulose, to some extent. Hemicellulose and amorphous cellulose have high free hydroxyl groups responsible for the great hygroscopicity of wood. Extraction of hemicellulose by steam or alkali solution increases dimensional stability (Fatrawana et al., 2019; Hornus et al., 2020). Additionally, alkali extraction dissolves part of lignin (Nawawi et al., 2021) which may contribute to the variation in wood crystallinity. The heat treatment carried out during densification is suspected of facilitating chemical properties alterations and can damage the hydrogen bonds between hemicellulose-lignin molecules (Amin and Dwianto, 2006). It also boosts weight loss and changes wood crystallinity, indicating components’ degradation (Kim and Kim, 2019; Kim et al., 2018).
In this research, changes in wood chemical composition during the densification process may occur due to the alkali pretreatment, temperature, or a combination of both variables. The statistical analysis showed that the boiling time significantly affected densified kapok wood ASE. Based on Duncan’s test, 3 h of boiling yielded the highest ASE values which were significantly different when compared to the 1 h result but not significantly different from 5 h. Further chemical composition analysis was conducted on the effect of these variables on densified wood with the highest ASE value. This aimed to determine the phenomenon of changes in chemical properties that are thought to affect the dimensional stability of alkali-pretreated and densified wood.
The dimensional stability of densified wood improved by alkali pretreatment was assumed to be the result of a chemical composition change. Table 1 data confirmed that 1.25 N NaOH treatment for 3 h was the main factor for the degradation of cell wall components particularly hemicellulose, and cellulose to a minor degree. However, lignin was relatively stable, and its higher measured value might be due to an increase in the proportion contained in the treated sample. The 100°C densification temperature only had a small effect on the change in densified woods’ chemical composition which was slightly lower when compared to the initial sample.
The holocellulose of kapok wood decreased by 21.81% through boiling in 1.25 N NaOH solution for 3 h, and the densified wood’s ASE value increased by 63.24%. Alkali solution degraded and dissolved hemicellulose and cellulose chemically during pretreatment. Also, heat tends to initiate components’ degradation during densification. Wood dimensional stability is related to the cell wall’s hydroxyl groups, which can form hydrogen bonds with water (Hon, 1996). Most hydroxyl groups are attached to hemicellulose and amorphous cellulose that are sensitive to degradation by alkali solution. This leads to a decrease in chemical components’ content in the alkali-treated wood, where the degradation of cellulose is smaller than hemicellulose. Cellulose is more resistant to degradation by alkali because it has a crystalline structure (Akgül et al., 2007).
In this research, lignin content was elevated due to alkaline treatment. Similarly, Kačíková et al. (2013) and Lukmandaru et al. (2018) suggested that the treated wood lignin increased because of the significant decrease in holocellulose content, even though there was probably a slight degradation of lignin. According to statistical analysis of variance, the densification and alkali pretreatment significantly affected the holocellulose and lignin content. Duncan’s test showed each treatment had a significantly different effect compared to the control.
The FTIR analysis confirmed the change in functional groups of alkali-pretreated and densified wood from comparison with the initial sample. The degradation of wood components (cellulose, hemicellulose, and lignin) may contribute to this change. The spectrum of FTIR analysis in Fig. 4 indicated that alkali pretreatment on densified wood did not change the functional groups but generated different absorption intensities.
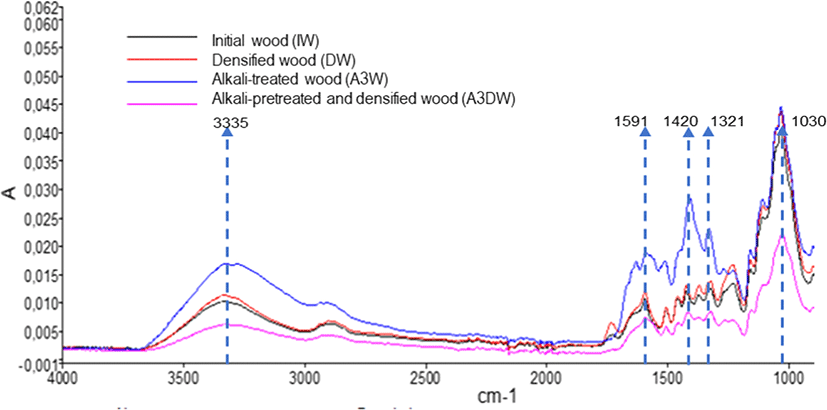
The absorption band at 3,334–3,335 cm–1 corresponds to the hydroxyl group (O-H) (Kim and Kim, 2019). In this wave number, the lowest absorbance value was found in the sample subjected to alkali pretreatment and densification, which reduced the hydroxyl groups and hygroscopic properties, as well as increased the dimensional stability. The change in the adsorption intensity of -OH groups was in agreement with the equilibrium MC of densified wood, hence both variables are directly proportional. Alkali treatment initially increased the hydroxyl groups, which is thought to be caused by the swelling of wood, and possibly the crystalline cellulose. The densification with pressure and heat facilitated the fixation between chemical components exhibiting the hydroxyl groups’ reduction.
In Fig. 4, absorption bands in the frequency range of 1,590–1,593 cm–1 indicated the aromatic skeletal vibration in lignin which was mainly detected by FTIR analysis in the wavenumber 1,505–1,594 (Chen et al., 2015; Naumann et al., 2007). The high absorbance in this range was found in alkali-pretreated wood. The sample’s relatively high proportion of lignin was initiated by the partial dissolution of holocellulose during alkali treatment. A higher proportion may contribute to greater dimensional stability, even in the presence of possibly lower crystallinity. This is due to the lignin being a plastic polymer with low hydroxyl group content. The same tendency was observed for the absorption band 1,420–1,421 cm–1 indicating the presence of C-H deformation in lignin and carbohydrates. Additionally, the C-O vibration detected in 1,230–1,321 cm–1 was for syringyl lignin, and 1,030–1,032 cm–1 was meant for cellulose and hemicellulose (Naumann et al., 2007).
FTIR data confirmed changes in the chemical composition of the alkali-pretreated and densified compared to densified woods. There was a decrease in the intensity of hydroxyl groups (absorption band at 3,335 cm–1) which coincided with a decrease in the vibration for cellulose and hemicellulose (absorption bands at 1,030 cm–1). Meanwhile, the change in lignin was relatively small as indicated by absorption bands at 1,420 and 1,321 cm–1. This confirmed that alkali pretreatment and densification caused changes in chemical composition, specifically holocellulose, and affected the characteristics of alkali-pretreated and densified wood to some extent.
XRD analysis measured the samples’ crystallinity. The CI describes the relative amount of crystalline moiety in wood. This increases with the elevation in the ratio of crystalline to amorphous part. Based on Table 2, the CI of alkali-pretreated and densified wood was lower than the control. Densification slightly decreased the crystallinity of the treated samples compared to the untreated due to applied heat. The exposure to 100°C temperature for 5 h during densification was suggested to promote partial component degradation, while alkali treatment caused a crystallinity decrease. Cellulose swelling and the increase in lignin proportion in the treated wood implied a decrease in the ratio of crystalline to amorphous part, as well as CI decline. This is based on the fact that lignin is an amorphous polymer in nature (Sjostrom, 1991). Accordingly, both alkali-pretreated and densified wood have a low CI.
No | Treatments | Crystallinity index (%) |
---|---|---|
1 | Initial wood (IW) | 73.11 |
2 | Densified wood (DW) | 62.90 |
3 | Alkali-treated wood (A3W) | 55.01 |
4 | Alkali-pretreated and densified wood (A3DW) | 39.21 |
4. CONCLUSIONS
Alkali pretreatment improved the dimensional stability of densified kapok. The different times of alkali pretreatment with 1.25 N NaOH solution significantly affected densified wood ASE. The highest ASE was obtained for alkali-pretreated and densified wood subjected to 3 h of boiling. The alkali pretreatment reduced holocellulose, particularly hemicellulose, and increased the relative lignin content which was in agreement with the results of FTIR and CI. The high dimensional stability of alkali-pretreated and densified wood was caused by the reduced hydroxyl groups and low equilibrium MC.