1. INTRODUCTION
The popular Pinus roxburghii, which belongs to the family Pinaceae, is locally known as “Salla”. Pinaceae leaves are long and needle-shaped, and the trees are evergreen conifers (Almendros et al., 2015). They are grown for commercial and industrial uses, such as soft timber, carpentry furniture, window frames, paneling floors, walls, and roofs, and some species are used as an important source of turpentine, pulp, or tar. Furthermore, different parts of these trees, such as barks, leaves, and cones are utilized for firewood (Hwang et al., 2021a). Therefore, Pinewood waste is a promising source of raw materials for producing porous carbon, which can be a novel material for energy storing devices.
Morphologically, Pinewood is composed of well-oriented microfibers with fiber cells that have distinctly mesoporous structures with tracheids that are used to transport water, ion, and oxygen during metabolism. Based on the chemical composition, these trees are also an abundant source of lignocellulosic carbonaceous materials (Mondal et al., 2017). They contain 3 major structural components, cellulose, hemicellulose, and lignin, that are widely distributed throughout vascular plants where they form the structural support system and play a vital role in creating porosity. The amount of each component depends on features like the species, age, and the part of the plant from which it is extracted (Fengel and Wegener, 1983; Schulz et al., 2021). However, Pinus roxburghii, being a softwood, contains 45%–50% cellulose, 25%–35% hemicellulose, and 25%–35% lignin (Fan et al., 1982). Additionally, these woods have also been utilized for the adsorption of impurities from water (Shen et al., 2021).
Herein, we report the use of wood waste from local carpentry shops to prepare activated carbon (AC) for use in energy storage devices, such as supercapacitors. ACs are incredible and essential energy storage materials because of their high conductivity, high surface area, high porosity, low cost, and availability (Iswanto et al., 2021; Shen et al., 2021). Supercapacitors are poised to replace batteries because of their superior properties, such as fast charge–discharge capability, long working lifetime, and safety record (Kim et al., 2019; Zhang et al., 2015). Therefore, modern portable electronic devices, electrical vehicles, military weapons, space equipment, and several electrical devices demand the use of supercapacitors (Chen et al., 2020; Liu et al., 2016). Many researchers have investigated the wide applications of supercapacitors prepared from different wood-based precursors using different activation processes (Mohamed et al., 2020; Wang et al., 2019; Zhang et al., 2019).
In supercapacitors, charge storage takes place at the surface of the electrodes either through adsorption of ions into the pores of the electrode materials (i.e., electric double layer capacity (EDLC) or nonfaradaic capacitive charge) or surface redox reactions at the interface (i.e., pseudocapacitive or faradaic capacitive charge; Aadil et al., 2020; Chen et al., 2020). EDLC-type electrode materials produce high power density and longer life cycle retention but have low energy density (Wei et al., 2020), while pseudocapacitive materials provide high energy density but have low cycle stability (Krishnamoorthy et al., 2020).
A recent trend in enhancing the capacity and life of supercapacitor materials is to combine both types of charge storage mechanisms (i.e., EDLC and pseudocapacitive), to yield a hybrid-supercapacitor (Liu et al., 2017; Wong et al., 2020).
ACs are used as negative electrodes (cathodes) in EDLC to accumulate energy (Galih et al., 2020; Wang et al., 2019). Recent studies report that higher charge storage capacities can be delivered by pseudocapacitive anodes, such as transitional metal oxides like MoO3 (Zhang et al., 2019), V2O5 (Guo et al., 2015), WO3 (Yun et al., 2019), TiN (Sun et al., 2020), Fe3O4 (Arun et al., 2019), FeOOH (Chen et al., 2016), and Fe2O3 (Zhang et al., 2020), than by carbon materials. Moreover, hematite (Fe2O3) is considered an efficient transition metal oxide for the preparation of supercapacitor electrode materials (Li et al., 2019) owing to its many advantages, such as cost-effectiveness, natural abundance, excellent physicochemical stability, minimal environmental impact, large theoretical capacitance (3,625 Fg−1), and a high potential for hydrogen evolution in aqueous solution. Irrespectively, Fe2O3 electrode material has serious disadvantages, such as poor electrical conductivity and large volume change, reduction in the rate capacity and lifespan due to volume expansion, restricting its applications in high-power storage devices. However, incorporating AC with metal oxides, such as Fe2O3, is an alternative way of improving its conductivity. This leads to better ion diffusion and enhanced overall electrochemical properties compared with that of the individual components because of the synergistic effect between the metal oxide (Fe2O3) and AC (Xuan et al., 2020; Yang et al., 2020).
Hence, in this research, AC prepared from locally available Pinus roxburghii wood waste (carpentry waste) was used as a precursor for preparing a hybrid-supercapacitor electrode material with Fe2O3. The AC prepared was characterized using different instrumental techniques, such as X-ray diffraction (XRD), Fourier transform infrared spectroscopy (FTIR), X-ray photoelectron spectroscopy (XPS), Raman spectroscopy, scanning electron microscopy (SEM), and Brunauer–Emmett–Teller (BET) theory to understand the effects on the surface morphology, functionality, and porosity that are essential for exhibiting admirable electrochemical behavior. Thereafter, the AC was electrochemically characterized using cyclic voltammetry (CV), galvanostatic charge/discharge (GCD), and electrochemical impedance spectroscopy (EIS).
2. MATERIALS and METHODS
Pinus roxburghii (Salla) wood waste was collected from indigenous carpentry shops in Kathmandu, Nepal. Analytical grade chemicals were used as received without further treatment. The activating agent for the preparation of AC was 85% phosphoric acid (H3PO4) with a specific gravity of 1.73 g/mL (15.0 M) obtained from Fischer Scientific, India. (P) Ltd. Double-distilled water was used throughout the experimentation. Carbon black, polyvinylidene fluoride (PVDF), and N-methyl pyrrolidone (NMP) were supplied by Sigma-Aldrich (St. Louis, MO, USA) and Fe2O3 was gotten from APS Ajax Finechem (Seven Hills, Australia). Additionally, Ni-foam was obtained from PRED MATERIALS, International (New York, NY, USA).
Firstly, the collected wood waste was sun-dried for a few days. In the preliminary stages, the sun-dried sample was air-dried, crushed, milled, ground, and screen-sieved. The particle sizes were controlled by sieving with a 150 μm size sieve to obtain a fine wood powder that was used as a precursor for the preparation of AC.
AC was synthesized using a previously reported protocol (Kim and Kim, 2020; Özcan and Korkmaz, 2019; Shrestha, 2021; Shrestha et al., 2019) in which the precursor was impregnated chemically with H3PO4 in a ratio of 1:1, i.e., the weights of the precursor and activating agent were 1:1 (w/w), and subsequently kept at room temperature for 24 h for proper soaking. Afterward, the resulting sample was dried using evaporation methods at 110°C for 2 h in an oven. Thereafter, the carbonization process was started by inserting the sample into a tubular furnace at 400°C for 3 h in a continuous N2 flow at 100 mL/min. Subsequently, the carbonized samples were cooled to room temperature by maintaining the N2 atmosphere. Afterward, the cooled samples were washed with hot distilled water severally to remove impurities fast and subsequently washed with cold distilled water till the measured pH was neutral. Finally, the samples were dried at 110°C (Hwang et al., 2021b; Lee et al., 2021; Shrestha and Rajbhandari, 2021), ground, and used for physical and electrochemical characterizations. The samples are represented as prepared activated carbon (Pr-AC).
An SDT Q600 V20.9 Build 20 USA thermogravimetric analyzer was used for the thermogravimetric analysis/differential scanning calorimetry (TGA/DSC) analysis of the raw wood powder (Pr-R). Pr-AC was characterized using different instrumental techniques: XRD (RIGAKU X-ray diffractometer, RIGAKU, Tokyo, Japan) was used to study the phase state of Pr-AC, and defects were examined using Raman spectra (labRAM HR800 France, JOBIN YVON, Edison, NJ, USA). The oxygen content of Pr-AC was inspected using FTIR (BRUKER-OPTIK GMBH, Ettlingen, Germany; Vertex 70/80, Bruker, Billerica, MA, USA) and an XPS (MultiLab 2000, Thermo Fischer Scientific, Waltham, MA, USA) system with an Al Kα source at 1.487 kV and 200 W. The surface area and pore volume were analyzed using the BET (Micromeritics ASAP 2020 system, Micromeritics, Norcross, GA, USA) method and SEM (Mini SEM nanoeyes, Tesko, Daegu, Korea) was used to identify the surface morphology of Pr-AC.
The synthesis of Pr-AC-electrode-material was as follows: A mixture of 8 mg of Pr-AC (powder), 1 mg of carbon black powder, and 1 mg of PVDF were ground in a mortar. Thereafter, 200 μL of NMP solution was added to the mixture to dissolve PVDF. The mixture was ground thoroughly and the resulting paste was the electrode material. Afterward, approximately 70 μL of the paste was applied to a rectangular-shaped Ni-foam of 1 cm2 area.
Similarly, hybrid composite electrodes (HCEs) were fabricated. The active materials for the hybrid composites were Pr-AC and Fe2O3 in 3 Pr to Fe2O3 mixed ratios of 1:1, 1:2, and 2:1. Finally, the fabricated electrodes namely: Pr-AC-electrode, HCEs (1:1, 2:1, and 1:2), and Fe2O3-electrode, were oven-dried at 70°C overnight, pressed individually at 10 kPa pressure for 1 min, and soaked overnight in 6 M KOH solution before electrochemical measurements. Thereafter, electrical contact with the electrode was made through a Cu wire.
The electrochemical performances of the fabricated electrodes were tested individually in a 6 M KOH solution using a 3-electrode experimental setup consisting of Pr-AC-electrode, HCEs, and Fe2O3-electrode separately as working electrodes, a Pt plate as a counter electrode, and Ag/AgCl as a reference electrode. The cell was connected to a Metrohm Autolab (PGSTAT 302 N) potentiostat/galvanostat system and the experiment was performed at room temperature.
A potential window of –1 to –0.2 V was chosen for CV measurement of Pr-AC-electrode and –1.15 to –0.4 V for HCEs, and varying scan rates of 2, 5, 10, 20, 50, and 100 mV s−1 were used. GCD testing was observed over the same potential window, employing current densities of 1, 2, 3, 5, 10, 15, and 20 Ag−1, and the cyclic stability (% retention) was estimated from the GCD analysis. A frequency range of 100 kHz to 0.1 Hz was used at a perturbation signal of 10 mV for EIS measurements, and a Nova 1.1 software was used to fit the EIS data (Ding et al., 2020; Shrestha, 2021; Shrestha et al., 2019).
3. RESULTS and DISCUSSION
TG/DSC analyses were employed to understand the thermal decomposition behavior of Pr-R as shown in Fig. 1.
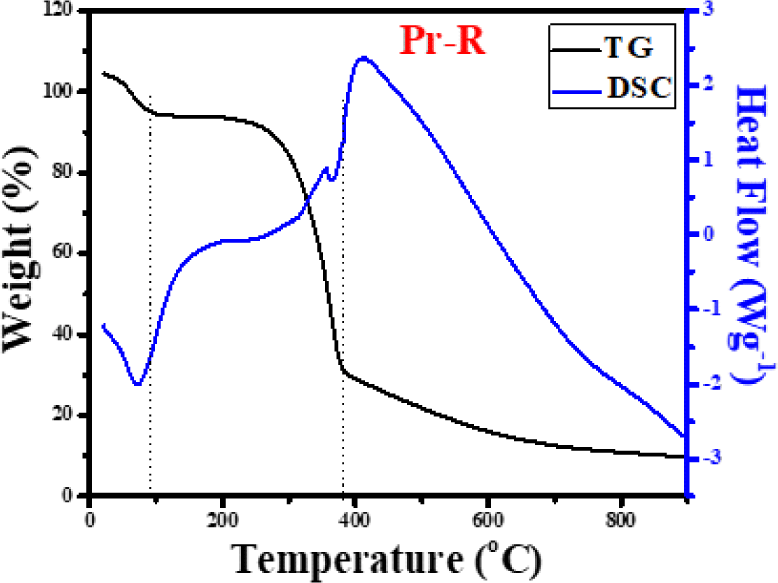
Fig. 1 shows that Pr-R has a slight weight loss around 100°C in the TG curve that may be due to moisture loss; this is verified by a sharp peak at 100°C in the differential scanning calorimetry (DSC) curve. Furthermore, there is a contracted peak between 200°C and 300°C which is not seen in the TG plot. However, slight weight loss is detected in the DSC curve between 200°C and 300°C that is attributed to the complete breakdown of hemicellulose between 300°C to 310°C (Gao et al., 2017; Hwang et al., 2021a). Between 300°C and 400°C on the TG curve, a noteworthy weight loss that is confirmed at 390°C in the DSC curve is seen. This means that cellulose starts to break down at 300°C and is transformed into organic volatile matter present in raw wood dust powder at 400°C. The TG curve shows that the wood dust powder is steadier beyond 400°C (Shrestha and Rajbhandari, 2021), whereas in the DSC curve depressions (endothermal peaks) are seen around 50°C and 390°C. No depressions are seen in other parts of the curve; hence, a heating rate of 400°C is postulated to be adequate for carbonization.
The amount of precursor left after carbonization at 400°C is approximately 50%, i.e., 50% is lost in the process as shown in Fig. 1.
The XRD profiles for Pr-AC are shown in Fig. 2(a). The broad diffraction peaks centered around 23.5° and 43.5° correspond to the (002) and (100) planes of AC (Gao et al., 2016), respectively. The amorphous nature of carbon is identified through the broad peaks (Molina-Sabio et al., 1995), and there is no observed sharp peak because of the absence of degree of graphitization (Liu et al., 2016; Wang et al., 2013). However, in some cases, insignificant peaks can be seen that may be due to the presence of impurities or moisture associated with the sample.
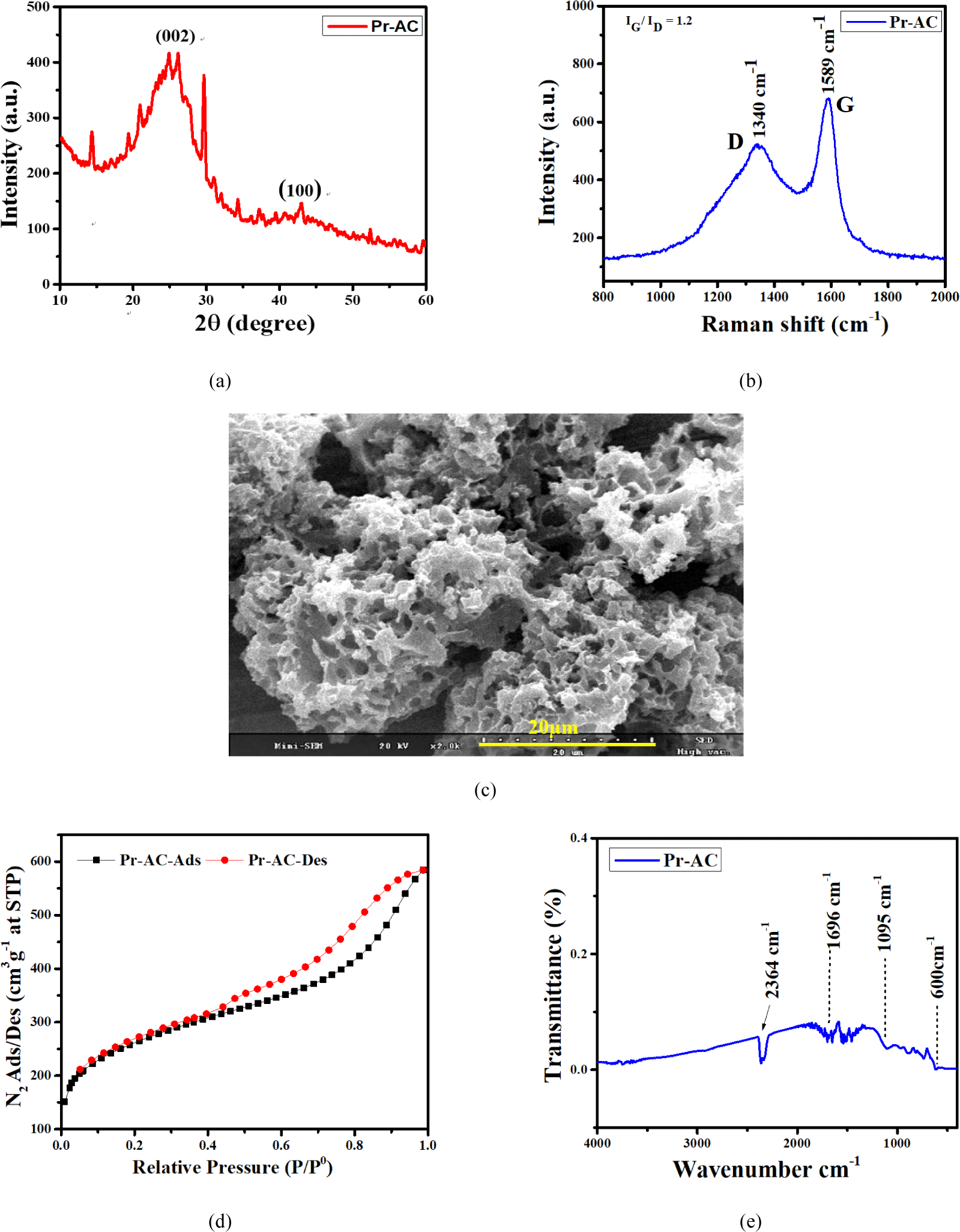
Raman spectra studies in Fig. 2(b) verify the amorphous nature of Pr-AC shown by the XRD profiles. Two relatively sharp peaks that are G and D bands at 1,589 cm−1 and 1,340 cm−1, respectively, are seen and the relative intensity ratio of the D band to the G band is approximately 1, indicating irregularity in the lattice space or a disordered carbon structure. Gao et al. (2017) reported that in contrast to crystalline materials, amorphous materials have a large number of vacancy-like structural defects (no regular atomic structure) that facilitate the diffusion of electrolyte ion and electrochemical reactions (Gao et al., 2016). Furthermore, amorphous materials experience isotropic stress and strain of charge–discharge that helps in better cycling stability.
SEM micrographs of the internal structure of Pr-AC at a magnification of x2.0k showing well-developed highly porous morphology with well-developed pores are illustrated in Fig. 2(c). The development of pores on the surfaces of Pr-AC is due to dehydration by H3PO4 and subsequent reaction with oxygen to generate phosphoric anhydride (P2O5). When P2O5 sublimated from solid to gas at a temperature of 360°C, gaseous P2O5 escaped by creating pores on the carbon surface (Ju et al., 2020; Sarkar et al., 2015). The remaining P2O5 was hydrolyzed and removed by micropores and mesopores creating vacant spaces equal to the amount of phosphoric acid removed (Xie et al., 2018).
The properties of the surface area and porosity of Pr-AC were evaluated using BET analysis [Fig. 2(d)] and the summarized results are presented in Table 1. N2 adsorption-desorption isotherms present type II behavior according to the Brunauer-Deming-Deming-Teller (BDDT) classification (Brunauer et al., 1938; Kondrat et al., 2012), indicating a strong presence of mesopores and a proportion of micropores.
AC | Specific surface area (m2/g) | Pore size (BJH Ads) (nm) | Pore volume (BJH Ads) (cm3/g) |
---|---|---|---|
Pr-AC | 916.23 | 3.96 | 0.69 |
The BET investigation discloses that in Pr-AC, the isotherms show a small nitrogen uptake at low pressure (P/P° < 0.1), signifying the existence of limited microporosity. Conversely, the amount of nitrogen uptake starts from the relative pressure (P/P°) of 0.4 and is augmented, displaying a hysteresis loop throughout the process of nitrogen adsorption and desorption of up to 1.0 (P/P°). This indicates the presence of enormous mesoporosity in Pr-AC. Such pores are also noticeable in the SEM image [Fig. 2(c)]. The results obtained from BET measurement are shown in Table 1.
Pr-AC exhibits a surface area of 916.23 m2/g, pore size of 3.96 nm, and pore volume of 0.69 cm3/g. The SEM micrograph of Pr-AC [Fig. 2(c)] shows a highly porous structure, and such porosity could be interrelated. The porosity may be attributed to the release of P2O5 during carbonization and the washing phases that generate immense pores (Pankaj et al., 2018). Porous carbon materials have the advantages of large specific surface area, high conductivity, stable structure, and relatively low price, making them ideal electrode materials for supercapacitors (Duan et al., 2020).
Fig. 2(e) displays the FTIR spectra of Pr-AC. The peak at 2,364 cm−1 corresponds to the –OH stretching of carboxyl, phenol, alcohol vibration, and adsorbed water. The existence of this peak indicates improved functionalization in raw wood dust after H3PO4 activation (Duan et al., 2020). The peak at 1,696 cm−1 is attributed to olefinic C = C vibrations in aromatic rings (Liu et al., 2016). The peak at 1,095 cm−1 is caused by the stretching of -C–O bonds in acids, alcohols, phenols, ethers, and esters (Li et al., 2020). The peak near 600 cm−1 is ascribed to C–C stretching in the fingerprint region. These show that plenteous oxygen-containing groups are formed on Pr-AC because of chemical oxidation. The FTIR results show that Pr-AC is abundantly functionalized with oxygen-containing functional groups, such as carboxylic, ether, and phenolic groups after H3PO4 activation.
The distributions of carbon and oxygen on the AC surface can be derived from the C 1s and O 1s spectra, respectively. To reveal additional evidence about the surface chemistry and binding natures of carbon and oxygen on Pr-AC, XPS spectra measurements were taken as shown in Fig. 3(a–c). Typical XPS survey spectrum of Pr [Fig. 3(a)] exhibits C 1s, O 1s, and P 2p peaks with no other detected elemental peaks, revealing that the Pr-AC comprises 3 main elements: C, O, and a trace amount of P.
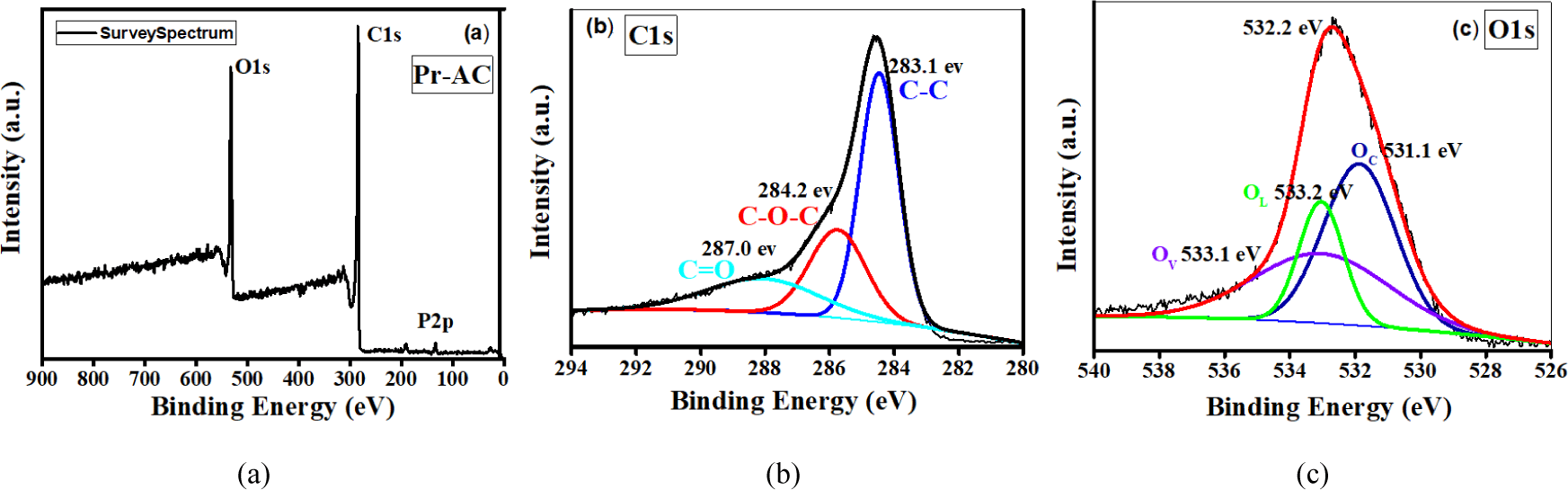
Fig. 3(b) shows the C 1s signals of Pr-AC. The maximum C 1s signal on Pr-AC shifts to higher binding energy due to an increase in oxidic species (alcohols and carboxylic groups) and ethers on the carbon surface after acid treatment (Li, 2012). Fig. 3(b) shows the broad peak of C 1s. On deconvolution, 3 peaks are positioned at binding energies (BE) of 283.1 eV for C–C which is for a nonfunctionalized carbon, 284.2 eV for ether and alcohol (R-O-R/-OH), and 287.0 eV for aldehyde and ketone (-CHO/C = O; Maile et al., 2019). Three deconvoluted oxygen peaks (O 1s) are assigned to phosphatic oxygen (PO43−;) at 531.1 eV, a hydroxyl group (-OH) at 533.2 eV, and silicon dioxide (SiO2) at 533.1 eV. The broad peaks observed indicate an overlap of various functional groups. Fig. 3(a) and (b) confirm that Pr-AC is finely functionalized after H3PO4 activation and comprises more acidic functional groups, such as aldehydes/ketones, ethers, and hydroxides (Maile et al., 2019). The results reveal that the breakdown of cellulose, hemicellulose, and lignin, cross-linking of H3PO4, and functionalization occur as a consequence of carbonization and activation. Carbon and oxygen are present in Pr-AC, and Wong et al. reported that when the carbon and oxygen-containing AC is used as an electrode material for a supercapacitor, it helps to increase the pseudocapacitance and cycling stability (Wong et al., 2020). Summarily, Pr-AC is amorphous, highly porous with enormous mesoporosity, and has a large surface area and oxygenated functional groups that are the required characteristics of ACs used as electrode materials. This motivated the application of Pinus roxburghii derived AC, Pr-AC, as electrode materials for supercapacitors.
The XRD pattern of the 1:1 hybrid composite of Pr-AC:Fe2O3 (Fig. 4) shows diffraction peaks for α-Fe2O3 at 24°, 34°, 36°, 42°, 50°, 54°, 57°, 63°, and 65°, corresponding to the (012), (104), (110), (113), (024), (116), (018), (214), and (300) planes, respectively. Wang et al. (2019) reported that the XRD pattern for pure α-Fe2O3 shows a main peak at 33°, corresponding to the (104) plane which is an indication of the formation of the Fe2O3 phase (Wang et al., 2009). All the peaks observed in Pr-AC:Fe2O3 show the formation of a homogenous composite and they are mostly lattice parameters of the composites calculated from the diffraction peaks at (104) and (110) planes that agree with JCPDS no. 89-8104 (Zhang et al., 2015).
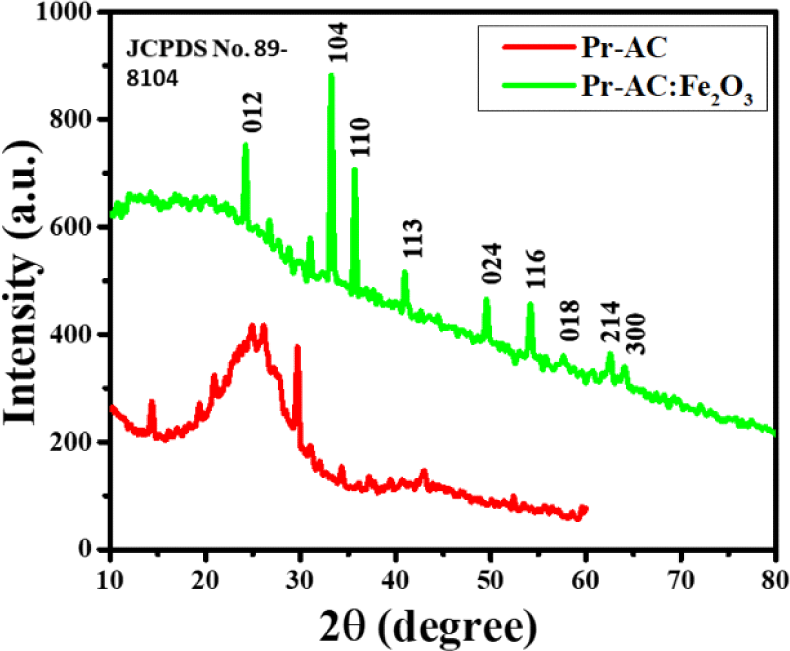
The CV curves of Pr-AC-electrode at different scan rates of 2, 5, 10, 20, 50, and 100 mV s−1 at potential windows of –1.2 to –0.1 V, –1.1 to 0.0 V (Figures not shown), and –1 to –0.2 V in 6 M aqueous KOH electrolyte were observed. Among them, the potential window of –1 to –0.2 V was most suitable based on the current density and shape of the CV curves [Fig. 5(b)]. The Pr-AC-electrode exhibited quasi-rectangular shapes in their CV curves without obvious distortion, indicating their reversibility. The high scan rate did not distort the features of the CV (Zequine et al., 2017).
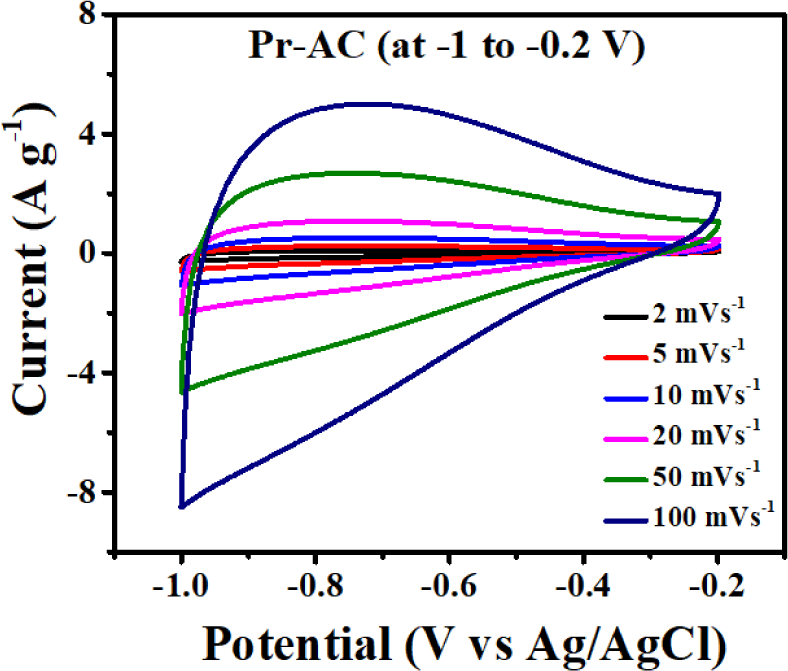
The CV curve of the Pr-AC-electrode at a scan rate of 100 mV s−1 shows performance similar to the rectangular cyclic performance of an ideal capacitor with no redox peak that enables easy and smooth passage of electrolyte ions. Hence, no redox peaks are observed in the CV curves, and the charge storage mechanism in Pr-AC-electrode seems to be due to the EDLC mechanism (Mondal et al., 2017). The current density is approximately 6 Ag−1. From Fig. 5, the negative potential limit can be fixed at –1.15 V as there is an increase in the current above this potential due to an H2 evolution reaction, which needs to be avoided. However, the value acquired from CV is not so noteworthy when compared with those from other studies (Puziy et al., 2002). Therefore, for the enhanced electrochemical performances of Pr-AC, its hybrid composite with Fe2O3 was studied, and its electrochemical capacitive behaviors were investigated.
Many potential windows were tried for HCEs; however, the potential window of –1.15 to 0.4 V was found to be the best, with all 3 (1:1, 1:2, and 2:1) HCEs displaying rectangular EDLC curves and redox peaks as shown in Fig. 6. Nevertheless, when the amount of Fe2O3 was doubled, the capacitance declined, which may be due to the accretion of the Fe2O3 particles inside the composite or due to the high bulk resistance value of pure Fe2O3 [Fig. 6(b); Chakraborty et al., 2020].
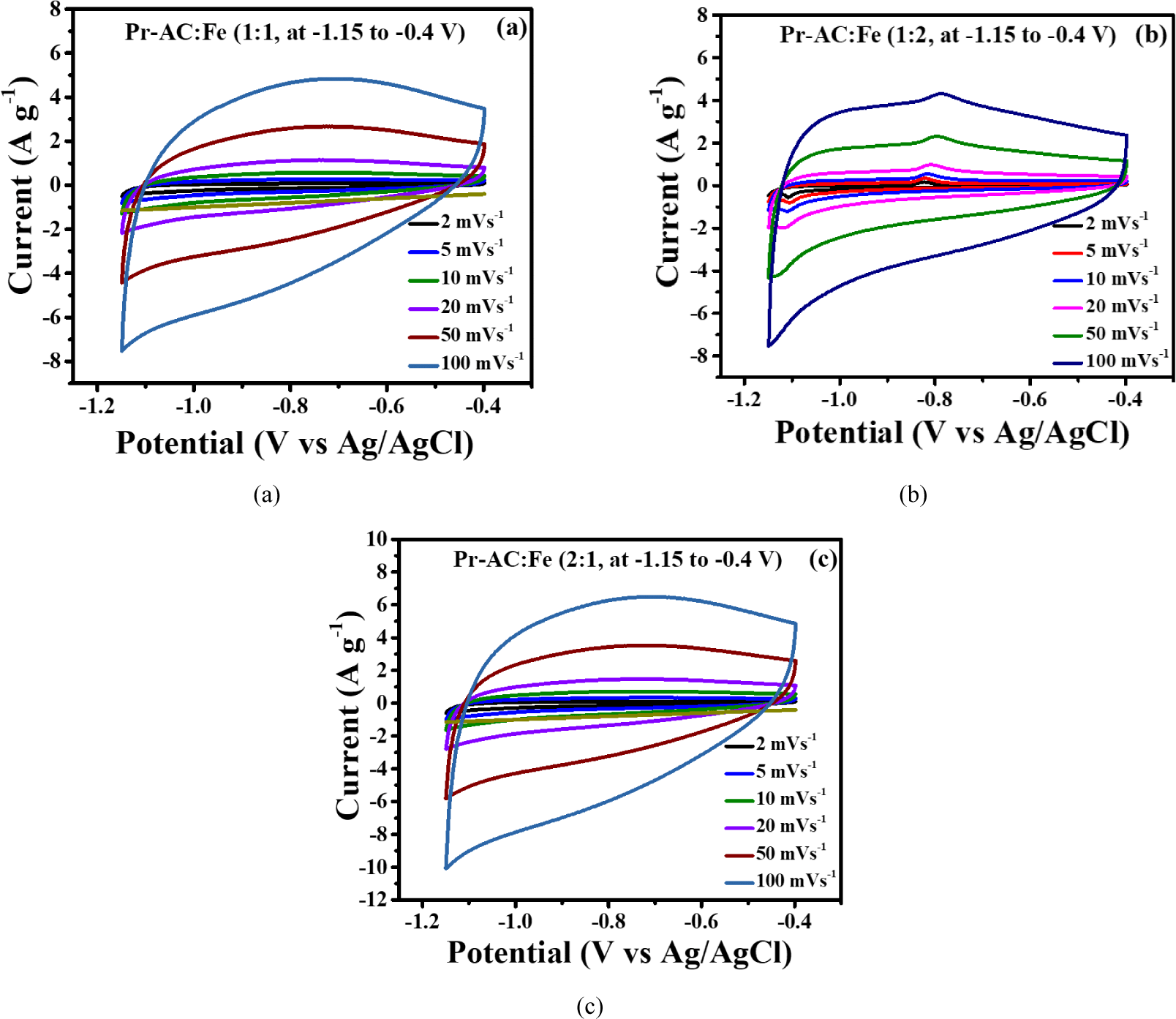
When the quantity of Fe2O3 was halved, the redox peak almost disappears and the rectangular shape of EDLC becomes dominant, as shown in Fig. 6(c). This demonstrates that the redox feature of the composite electrodes practically depends on the quantity of Fe2O3 since redox reactions take place on Fe2O3. The redox reactions are due to the reversible conversion of Fe3+ to Fe2+ (Chakraborty et al., 2020; Kim and Kim, 2020); therefore, introducing Fe2O3 into the electrode material imparts pseudocapacity. However, in 1:1 (Pr-AC:Fe2O3), the redox behavior seems moderate which may be due to the saturation of Fe2O3 particles within the composite.
The electrochemical properties of Pr-AC-electrode are studied further using a GCD process at the same potential window of –1 to –0.2 V as shown in Fig. 7.
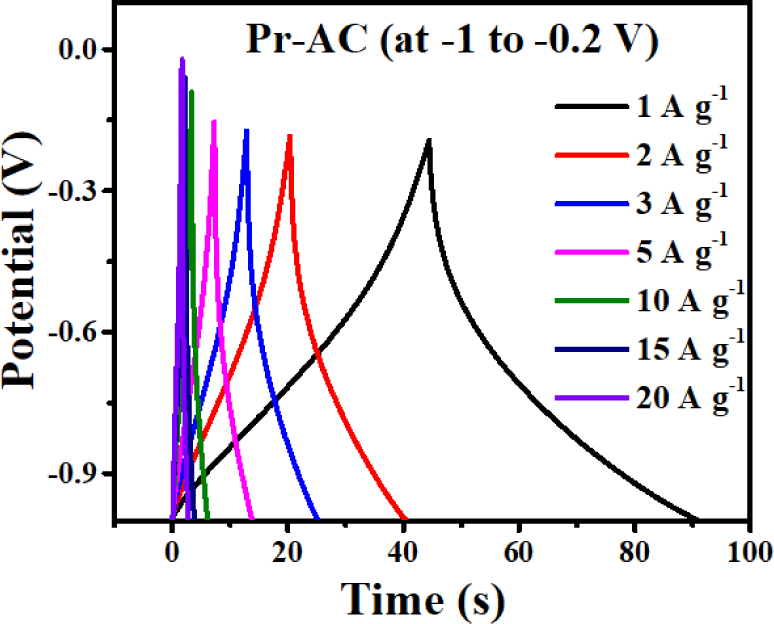
Fig. 7 shows that at a low current density the GCD curve is nearly triangular; however, the curve still shows symmetry, indicating the high charge–discharge reversibility of the sample material.
Afterward, the HCEs (Pr-AC:Fe2O3) were studied further using a GCD process in the –1.15 to –0.4 V potential window. The GCD curves for HCEs are shown in Fig. 8(a–c). The GCD curves have an approximately triangular shape at low current density with a bending nature due to redox reactions in the pseudocapacitor material (Xuan et al., 2020). Irrespectively, the GCD curve retains symmetry owing to reversibility in the charge–discharge behavior of the material. The discharge time for the composites decreased with increasing amounts of Fe2O3 (70 s for 2:1, 60 s for 1:1, and 50 s for 1:2).
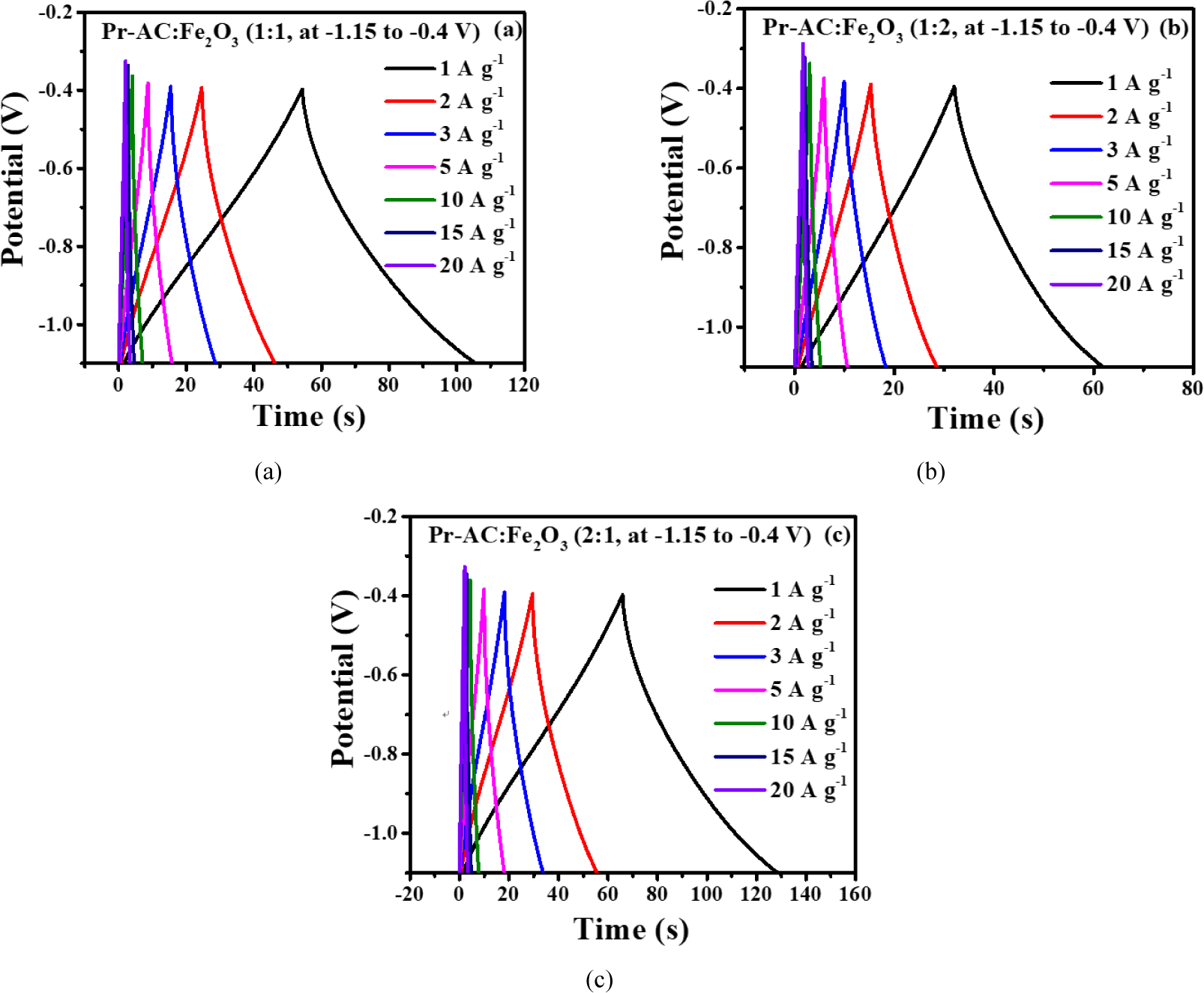
The specific capacitances of all the fabricated electrodes were calculated using Equation (1) (Sarangapani et al., 1996):
where I (A) is the discharge current, Δt (s) is the discharge time consumed in the potential window of ΔV(V), and m (g) is the mass of active material.
The specific capacitance of Pr-AC-electrode was 59.02 Fg−1. However, the specific capacitances of hybrid composites were higher at 102.2 Fg−1 for the 2:1 ratio, as shown in Table 2. The better specific capacitance value of 2:1 HCEs compared with that of the Pr-AC-electrode may be due to the homogenous distribution of Fe2O3 on porous Pr-AC or low resistance, which is further discussed with the EIS analysis.
The effect of current density on the specific capacitance is displayed in Fig. 9. The curve shows a sharp decline in the specific capacitance at a current density of up to 10 Ag−1 in all electrodes except 1:2 HCE; thereafter, the current density is approximately constant. At 1 Ag−1, 1:2 HCE showed the lowest specific capacitance of 49.04 Fg−1, while 2:1 HCE showed the highest of 102.22 Fg−1, showing more than double enhancement of the specific capacitance. At 20 Ag−1, the enhancement of the specific capacitance is only about 1.4 times because the higher current density limits the diffusion of ions into the inner pores of the electrodes, leading to a lowering of the specific capacitance (Lee and Goodenough, 1999). The specific capacitances of Pr-AC-electrode and HCEs at different current densities are shown in Table 2.
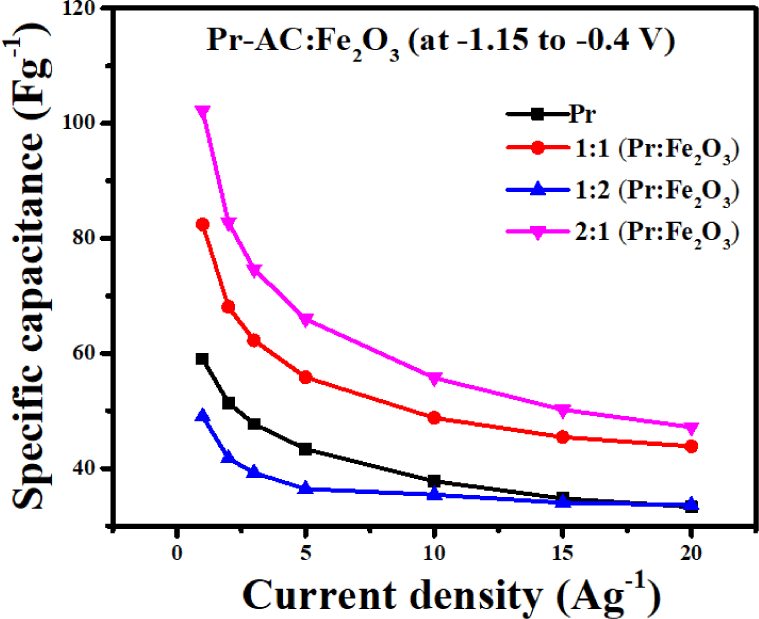
The life cycle or stability of the fabricated electrodes was investigated using capacity retention (%). In this study, 1,000 continuous charge–discharge cycles were applied at a current density of 3 Ag−1. The comparison of the capacity retention (%) of Pr-AC-electrode, (Pr: Fe2O3)-HCEs, and Fe2O3-electrode are shown in Fig. 10.
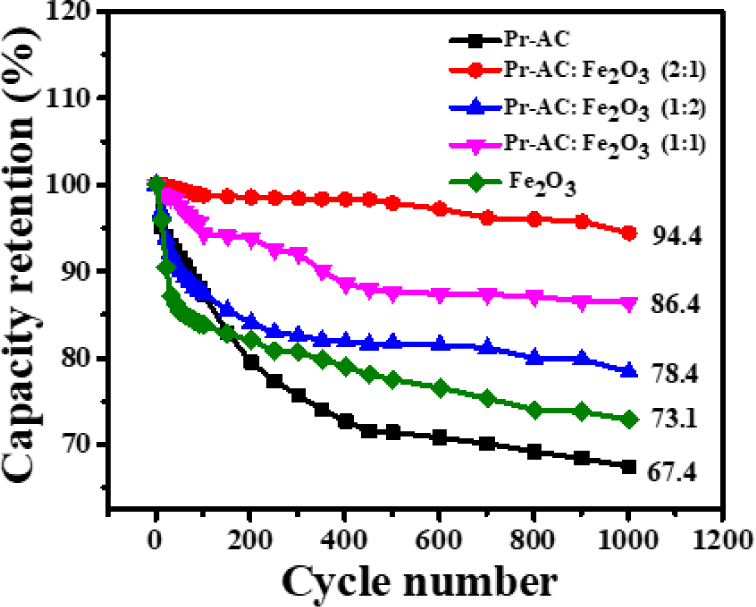
Fig. 10 shows that the Pr-AC-electrode has a poor retention capacity of 67.4%. However, the benefit of compositing the Pr-AC-electrode with Fe2O3 in the 2:1 ratio is noticeable from its high retention capacity of 94.4%. Contrarily, the 1:1 and 1:2 Pr-AC:Fe2O3-HCEs show somewhat low retention capacity than the 2:1 composition. This can be explained by the presence of a higher amount of Fe2O3 which exhibits a low retention capacity of 73.1% at the end of 1,000 charge–discharge cycles. The lower retention capacity of Fe2O3 is due to its high equivalent series resistance (ESR) value. The best retention capacity of 2:1 Pr-AC:Fe2O3-HCEs indicates electrochemical stability of the material due to excellent electrical conductivity (least ESR among all) and low degradation of the composite (Shrestha, 2021; Shrestha and Rajbhandari, 2021; Shrestha et al., 2019). The cycling of potential results in the degradation of electrode materials and causes an increase in the ESR value, lowering the capacity retention (Maile et al., 2019).
EIS measurements for Pr-AC-electrode, (Pr-AC:Fe2O3) -HCEs, and Fe2O3-electrode were carried out to analyze the resistive component in the system, and the results are depicted in Fig. 11.
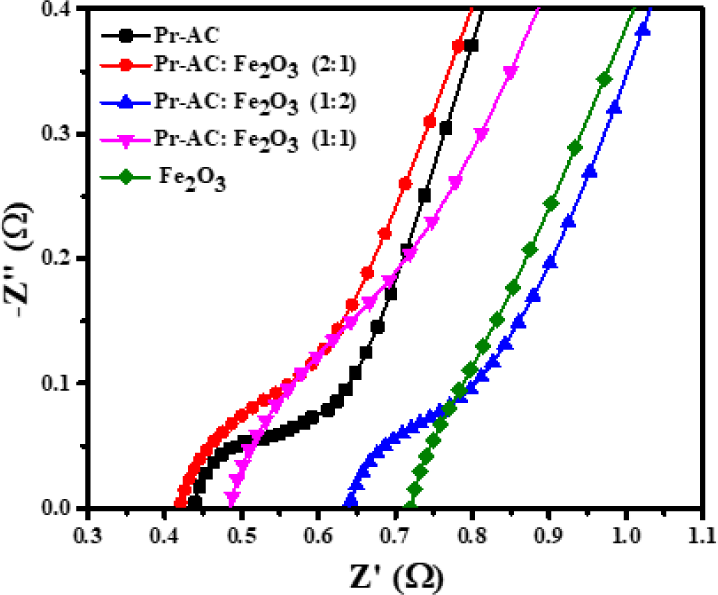
Fig. 11 shows that in high-frequency regions a semicircular loop is formed from which the ESR can be estimated as the total ESR due to electrode and electrolyte. A straight line at the low-frequency region is due to diffusion and transportation of electrolytes into the porous site on the electrodes.
The different ESR values in the Nyquist plots display the effect of the ratios of the composite materials. The results show that compositing Pr-AC-electrode visibly increases its ESR value; the 2:1 composite has the least ESR value of 0.42 Ω while the 1:2 composite has the highest of 0.64 Ω. The ESR value for Fe2O3-electrode is higher (0.73 Ω) than that for the (Pr-AC:Fe2O3)-HCEs as seen in Fig. 11. Therefore, the composition of the electrodes is a chief feature in developing the resisting component of the electrode.
The Ragone plots of all fabricated electrodes evaluated from GCD measurements at a potential window range of –1.15 to –0.4 V are shown in Fig. 12. The energy and power densities of all fabricated electrodes are calculated using Equations (2) and (3) and the results are presented in Table 3.
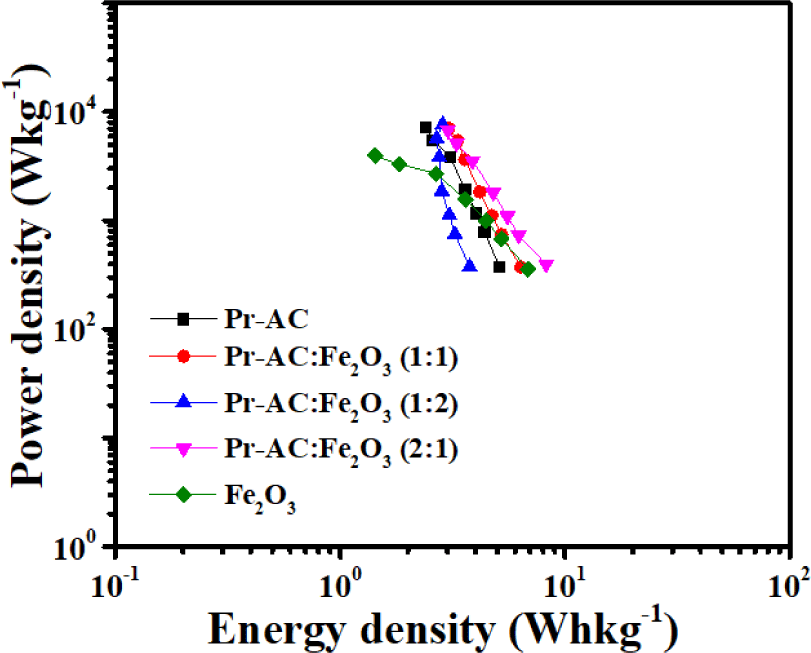
where E is the energy density in Whkg−1, P is the power density in Wkg−1, CSP is the specific capacitance in Fg−1, ΔV is the potential window, and Δt (s) is the discharge time. In this study, a 3-electrode system was used to measure the electrochemical performances, and the energy density was derived from dividing the CSP by 8 instead of 2.
The calculated energy density for the 1:1, 1:2, and 2:1 hybrid composites (Pr-AC:Fe2O3) were 6.46 Whkg−1 at a power density of 372.75 Wkg−1, 4.3 Whkg−1 at a power density of 373.88 Wkg−1, and 8.29 Whkg−1 at a power density of 395.15 Wkg−1, respectively, which are higher than the value for Pr-AC which is 5.12 Whkg−1 at a power density of 371.33 Wkg−1. Fe2O3 having pseudocapacitive behavior resulted in the enhancement of the energy and power densities through a fast and reversible faradaic process (Maile et al., 2019). The layered Fe2O3 structure allows intercalation and deintercalation of alkali metal ions (K+) during redox reactions between the Fe3+ and Fe2+ oxidation states. The surface adsorption mechanism of K+ on Fe2O3 may also be another probable reason for the enhanced energy and power densities in composites (Wei et al., 2020).
The results of specific capacitances, energy densities, power densities, capacity retention (%), and ESR values of Pr-AC-electrode, (Pr-AC:Fe2O3)-HCEs, and Fe2O3-electrode are summarized in Table 3.
4. CONCLUSIONS
In this study, Pinus roxburghii wood-waste derived AC was successfully prepared and characterized as supercapacitor electrode material. AC presented a BET-specific surface area of 916.23 m2g−1 and heterogeneous surface chemical composition. This heterogeneity was supported by XPS analysis results, suggesting the presence of oxygenated functional groups. A simple strategy was used to fabricate hybrid-nanocomposite electrode materials from AC and Fe2O3, and the electrochemical performances of the electrodes were evaluated. Due to the synergistic effect of AC and Fe2O3, the hybrid-nanocomposite electrode materials displayed optimum performance as supercapacitor electrode materials with an admirable capacity of 102.42 Fg−1 at a current density of 1 Ag−1, cycling stability retaining 94.4% capacitance after 1,000 cycles (at a current density of 3 Ag−1), and an energy density of 8.34 Wh/kg at a power density of 395.2 Wkg−1 in 6 M KOH electrolyte in a 3-electrode experimental setup, and a high working voltage of 1.55 V for 2:1 (Pr:Fe2O3)-HCEs.