1. INTRODUCTION
Drug delivery system (DDS) is a route of administration and the type of the drug to deliver the appropriate amount of the drug in a way that minimizes adverse effects and maximizes its efficacy (Rivas et al., 2017). To do so, it needs to enhance stability and efficiency by controlling spatio-temporal variability of drug efficacy by an improved drug delivery system. When the hydrogels absorbed large amounts of water, they behave like living tissues. Because they form a 3D scaffold with physical and chemical cross-links, this characteristic allows application of drug delivery system with high biocompatibility (An et al., 2017; Valle et al., 2017; Tanpichai and Oksman, 2016; Xiao and Zhou, 2013; Li and Mooney, 2016; Hyon et al., 1989; Pal et al., 2016). However, a low tensile strength of the hydrogels limits its application in parts that requires structural bearing power and decomposes quickly at a target point through an early melting process.
Also, it has been pointed out as disadvantages for industrial use of hydrogels that it is hard to load drugs in a hydrogel, which has a high water content and a porous structure, and it has a rapid release due to osmotic pressure (Rivas et al., 2017; McKenzie et al., 2015). For those reasons, some studies have been conducted by molding hydrogels, combining in various ways to improve mechanical strength (Wang et al., 2010; Hyon et al., 1989), and preparing a membranetype with a multi-layered structure to facilitate hydrophobic compounds binding to the internal cavity (Ladet et al., 2008; Lin et al., 2006; Zawko et al., 2008).
CNCs, which are organic-based nanomaterials produced using cellulose widely present in nature, were added to biodegradable PVA and applied to a hydrogel system. It is expected to improve the hydrogel's mechanical properties, increase the loading efficiency of hydrophobic drugs, and show a delayed release effect. The CNCs produced by acid hydrolysis are derived from sulfuric acid, and it features a length and thickness of 300 nm and 5-70 nm or less, respectively, resulting in a high surface area–to–volume ratio. It is easier to modify chemically than cellulose nanofiber (CNF), also based on nanocellulose, with a higher crystallinity and a higher reactivity due to many hydroxyl groups (-OH) on the surface. Therefore, it has been spotlighted as an eco-friendly reinforcement in the high-molecule composite material field (Gwon et al., 2016; Gwon et al., 2018; Khanjanzadeh and Park 2020; Zaini et al., 2019). In particular, CNCs can absorb large amounts of water due to their high specific surface area and numerous hydroxyl groups, and the sulfate (SO42-) formed on the surface by sulfuric acid treatment can maximize the efficiency of adsorption and delayed release of hydrophobic drugs. Therefore, application of CNCs is expected to be a good solution in terms of improving the mechanical properties and drug delivery efficiency of hydrogels.
In this study, CNC was applied to the PVA hydrogel system in which glutaraldehyde (GA) was used as a crosslinking agent, and the adsorption and release behavior of the drug was examined after salicylic acid (SA) was introduced as a drug model. To evaluate the structural properties of the hydrogel, fourier transform infrared (FT-IR) spectroscopy, water content, swelling ratio, specific surface area, scanning electron microscopy (SEM) and viscoelastic properties were analyzed. In addition, Langmuir, Freundlich adsorption model was applied to see interaction between hydrogels and the drug, and Lagergren's pseudo 1st order model and Ho's pseudo 2nd order model were applied for the release behavior.
2. MATERIALS and METHODS
In this study, hydrogel was prepared by adding CNCs, a crystalline polymer, to PVA (Mowiol® 10- 98, Sigma-aldrich), having a molecular weight of 61,000 Da, and glutaraldehyde (GA, Sigma-aldrich) was used as a crosslinking agent. The CNCs used in this study are made from cellulose powder (KC Flock W-50, average size: 45 μm) purchased from Nippon Paper Chemicals Co., Ltd. (Tokyo, Japan). And then, by the same method as in the previous study (Gwon et al., 2016; Gwon et al., 2018), the hydrolysis treatment was performed with 64 wt.% sulfuric acid (H2SO4, Junsei, Japan) for 20 minutes at 45°C, followed by washing with water until the supernatant became neutral by centrifugation. The CNCs/PVA suspension with distilled water used as a solvent was adjusted to pH 4.0 ± 0.2. GA was added and 0.5 N HCl (0.5N-Hydrochloric acid standard solution, Daejung Chemicals & Metals Co., Ltd, Korea) was used as a catalyst to induce binding reaction of pH 2.0 ± 0.02, preparing the hydrogels. And then, drug loading and release characteristics of the hydrogel prepared using salicylic acid (salicylic acid, ≥99.0%, Sigma-aldrich), one of the hydrophobic low-molecular-weight drugs, were observed.
The suspension was prepared as follows: After double-boiling 5wt.% PVA hydrogels with 200 mL of distilled water at 70 °C to melt, it was prepared with varying CNCs loading corresponding to 0, 10, and 20 phr of the dry weight, and stirred at 200 rpm for 6 hours. It was marked as CNC0, CNC10, and CNC20 depending on CNCs content. Each suspension having a different CNCs content was sufficiently stirred to prepare CNCs-PVA suspension with the CNCs were evenly distributed, and cooled in a desiccator to room temperature. GA of 5 phr (part per hundred resin) to PVA was added in the dripping method to the CNCs/PVA suspension and stirred for 5 minutes. After pH was adjusted to 2.0 ± 0.02 with 0.5 N HCl added, it was cured for 48 hours at room temperature in a 20 mm (d) × 8 mm (h) aluminum mold. The surface of the prepared hydrogels was washed with distilled water to remove unreacted cross-linking agents remaining on the surface after synthesis reaction and 0.5 N HCl used for pH adjustment until the surface turned neutral, and the finished specimens were stored in a chamber at 5 °C for use. The hydrogels were prepared in the same composition as described in Table 1. Fig. 1, shows that transparency decreased as the CNCs content increased, and no color change occurred.
Sample name | Component | |||
---|---|---|---|---|
PVA (wt%) | CNCs (phr) | GA (phr) | pH | |
CNC0 | 5 | 0 | 5 | 2.0±0.02 |
CNC10 | 5 | 10 | 5 | 2.0±0.02 |
CNC20 | 5 | 20 | 5 | 2.0±0.02 |
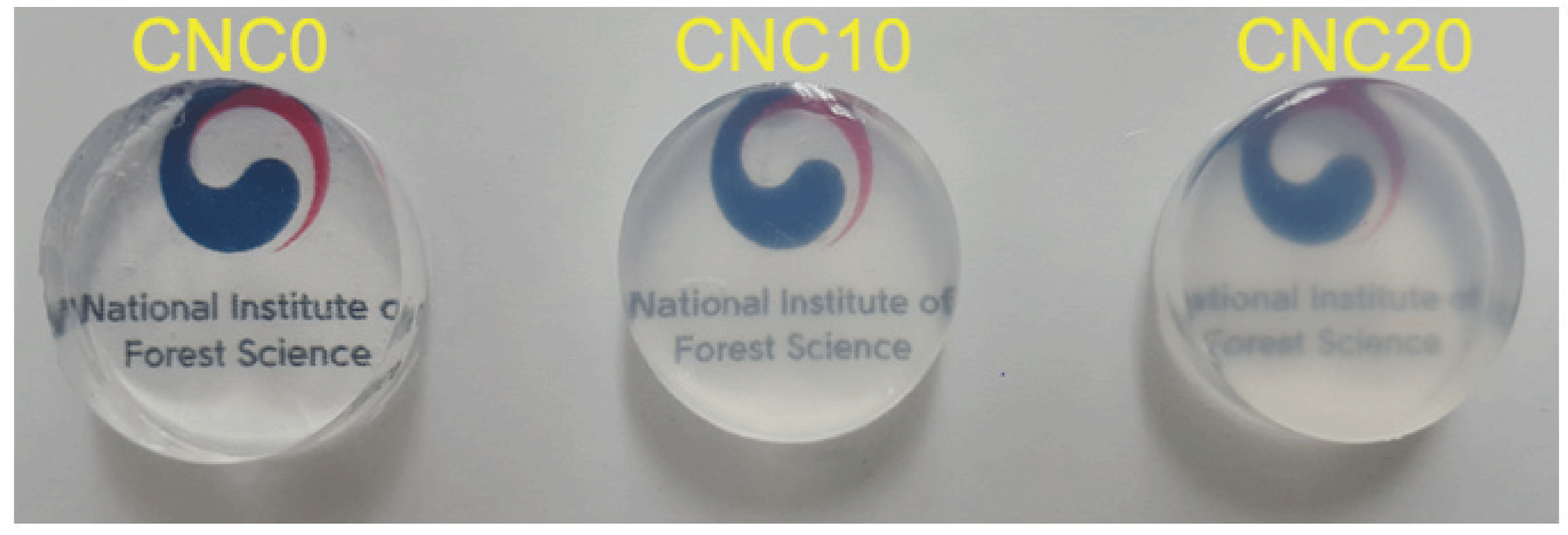
The prepared hydrogels were rapidly frozen under a liquid nitrogen atmosphere, stored in a freezer at –60 °C, and dried using a freeze dryer (FDU-1200, Eyela, Tokyo, Japan). The freeze-dried specimen was degassed at 20 °C for 30 minutes to remove residual moisture, and then specific surface area was measured with the nitrogen adsorption/desorption method (ASAP 2420, Micromertics®, United State) for 5 hours with liquid nitrogen.
The surfaces of the freeze-dried specimens were coated with thin gold films of 2 nm (Ion-Coater (KIC-IA), Coxem, Korea) by sputtering for 200 seconds at 4 mA, measured using FE-SEM (Coxem-30, Coxem, Korea), and different hydrogel images were obtained with different CNCs content.
An infrared spectrophotometer FT-IR (Nicolet iS10 FT-IR Spectrometer, Thermo Scientific, United State) was used to verify interfacial properties of the hydrogels with drug loaded and the ones without drug. All samples were used after surface was washed and dried for 24 hours in a chamber at 70 °C. However, the drug-loaded hydrogels were immersed in the drug for 48 hours in a chamber at 37 °C before beingwashed with distilled water to remove residuals on the surface until the hydrogel surface was neutral. FT-IR analysis was conducted in the ATR mode, the scan range of 400~4000 cm-1, the total scan of 32, the resolution of 8 cm-1.
To confirm the water content of the CNCs-based PVA hydrogels, thoroughly washed hydrogels were placed in a chamber at 70 °C, completely dried for 24 hours, and the weight difference was measured before and after drying using an analytical balance (HR-250AZ, A&D Co., Ltd., Korea). The weight was measured three times to be determined with the error range of ± (0.1%).
Wi: Initial weight of hydrogels
Wd: Weight of dried hydrogels
To check swelling properties, the hydrogels dried for 12 hours in a chamber at 70 °C were immersed in distilled water at 37 °C. The purpose was to check the swelling ratio according to the weight by time compared to the initial weight. The weight was measured three times to be determined in the error range of ± (0.1%).
Wd: Weight of dried hydrogels
Ws: Weight after swollen
To evaluate mechanical properties of hydrogels by CNCs content, CNCs-based PVA hydrogels’ storage modulus and loss modulus were analyzed using parallel and peltier plates with DHR3 rheometer (TA instruments, US). It was measured in the range of 0.1~100 radius/s for frequency sweep, 0.5 μN of axial force, and 10% of strain at 25 °C.
The storage modulus and loss modulus were determined with the following equation (Wang et al., 2010).
σ : Shear stress
ε : Shear strain
Salicylic acid, which is one of hydrophobic low-molecular- weight drugs, was used to study the same type of drugs that have difficulties in fast release and loading. For the loading experiment, the hydrogels immersed in distilled water for 48 hours in a chamber at 37 °C and swollen to 20 ± 2 Ø diameter, 8 ± 2 mm height, and 1.45 ± 0.1 g weight were impregnated with 200, 500, 1000, and 2000 mg/L of different concentrations of the drug. The amount of loaded drug was measured by HPLC (High Performance Liquid Chromatography, 1260 Series, Agilent).
The amount of the drug loaded per unit weight of CNCs-based PVA hydrogel was calculated with the following equation.
V: Volume of drug solution (mL)
w: Weight of hydrogel (g)
Ci: Concentration of initial drug (mg/L)
Ce: Concentration of drug at equilibrium (mg/L)
qe: Concentration at equilibrium after loading of the drug per unit weight of hydrogels (mg/g)
In order to obtain process parameters for applying the drug-loaded hydrogels to the drug system by the impregnation method, the appearance of the hydrogel interface and drug loading were predicted using an adsorption isotherm model. The interfacial reaction between the hydrogels and the drug was observed by using Langmuir isotherm, which is composed of a monolayer on a single interface and mainly shown in chemical bonds, and Freundlich isotherm, where a drug combines on a heterogeneous interface with multilayers by physical adsorption (Cho et. al., 2013).
Langmuir isotherm is as follows:
qe: Concentration at equilibrium after loading of the drug per unit weight of hydrogels (mg/g)
Ce: Concentration of drug at equilibrium (mg/L)
qmax: Maximum amount of the drug per unit weight of hydrogels (mg)
b: Langmuir adsorption constant
Langmuir isotherm is as follows:
qe: Concentration at equilibrium after loading of the drug per unit weight of hydrogels (mg/g)
Ce: Concentration of drug at equilibrium (mg/L)
KF: Freundlich adsorption constant
n: Adsorption constant from Freundlich isotherm
To verify the release behavior of the drug, the following environment was provided: the hydrogels were exposed for 48 hours to the condition where the drug concentration was 2000 mg/L to load them completely; the hydrogels loaded with the drug to have 20 ± 2 mm diameter, 8 ± 2 mm height, and 1.45 ± 0.1 g weight were completely immersed in 10 mL of distilled water in a chamber at 37 °C, which is similar to the body temperature, and stirred at 50 rpm so that the drug could be sufficiently released. HPLC (High Performance Liquid Chromatography, 1260 Series, Agilent, United State) was used to evaluate the concentration of the drug released per unit weight by time.
Pseudo 1st order model and pseudo 2nd order model were applied to dynamically analyze the drug release properties in drug-loaded CNCs-based PVA hydrogels over time.
Pseudo 1st order equation is as follows:
qe: Released amount during equilibrium (mg/g)
qt: Released amount per unit time (mg/g)
k1: Rate constant of the pseudo-first-order model
t : Reaction time
Pseudo 2st order equation is as follows:
qe: Released amount during equilibrium (mg/g)
qt: Released amount per unit time (mg/g)
k2: Rate constant of the pseudo-second-order model
t : Reaction time
Additionally, the initial release rate (h) with h = k2qe2 was obtained from the pseudo 2nd order equation (Cho et al., 2013).
Salicylic acid was analyzed by HPLC as described in Table 2.
3. RESULTS and DISCUSSION
The FT-IR spectra of the prepared CNCs-based PVA hydrogels loaded with the drug is shown in Fig. 2. As expected, the peak intensity of the 3000-3600 cm-1 region representing a free-OH group increased due to the addition of CNCs. Also, a -CH stretching vibration band in the 2930 cm-1 range, a C-H stretching band in the range of 2750 ~2850 cm-1 that are peaks seen in the aldehyde group, and a C=O vibration band in the range of 1720 to 1740 cm-1 appeared (Reis et al., 2006; Mansur et al., 2008; Hendrawan et al., 2019; Marin and Rojas, 2015). In particular, unloaded hydrogels showed the C=O groups that did not react to the cross-linking agent in the range of 720 to 1740 cm-1, but the peak intensity of loaded hydrogels decreased near 1700 cm-1 (Thangprasert et al., 2019). Also, peaks of acetal and ether groups formed by cross-link between the aldehyde group (-CHO) of GA and the hydroxyl group (-OH) of PVA and CNCs are shown in the regions of 1371 cm-1, 1250 cm-1, and 950 cm-1 (Yue et al., 2016; Yeom and Lee, 1996; Kim et al., 2003). The presence of the aldehyde group implies that there is an incomplete hemi-acetal linkage where only one of the two functional groups of GA is combined. Although the hemi-acetal group exists, the formation of the acetal and ether groups shows that the hydrogels prepared in this study using one type of polymer formed a 3D cross-linked network (semi- IPN) (Yue et al., 2016).
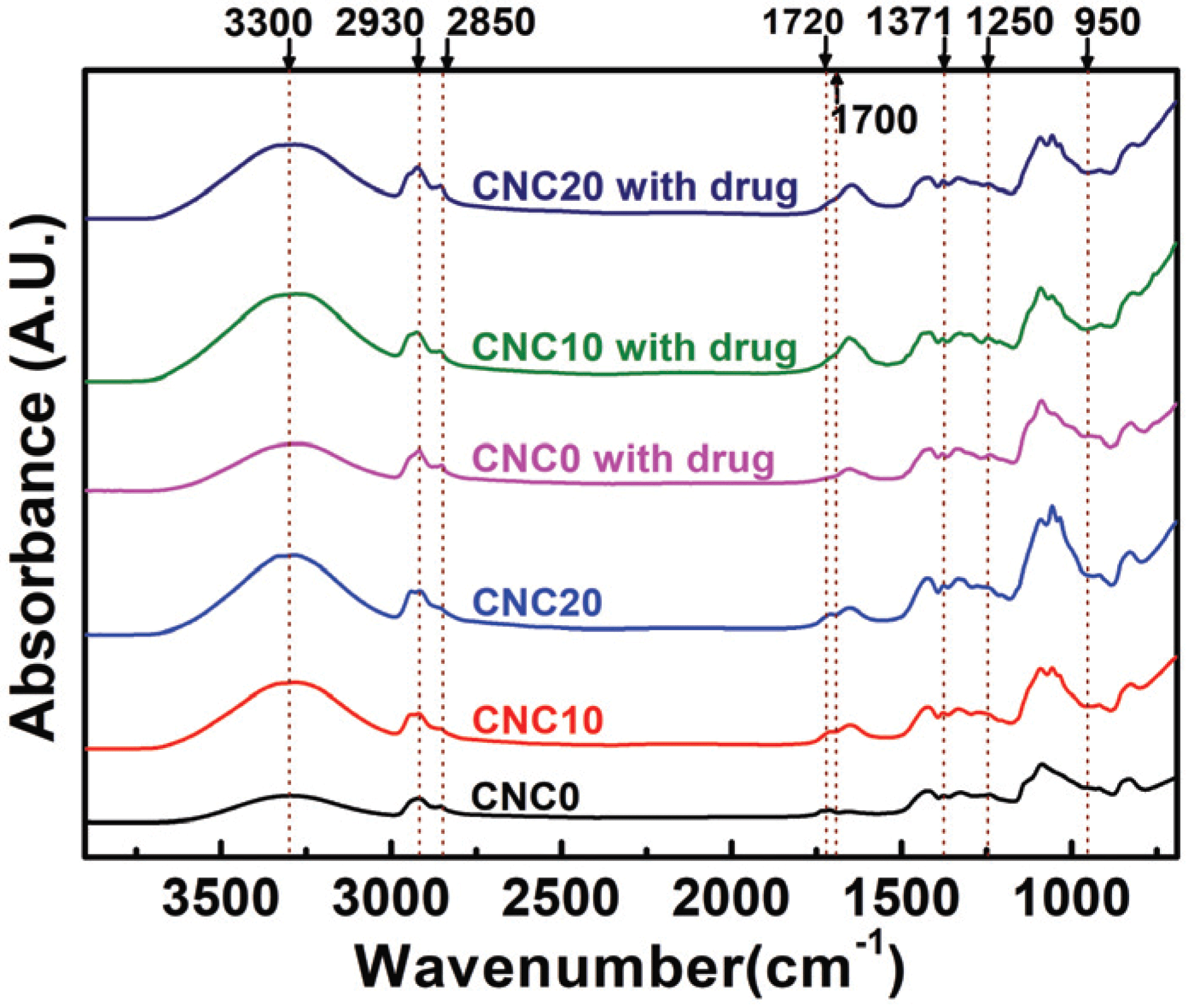
Fig. 3 shows SEM images that were measured to verify the 3D scaffold network (semi-IPN) of the hydrogels. From these results, it was concluded that PVA, CNCs and GA had a 3D pore structure interconnected by crosslinking between hydroxyl groups, and the size of the pores increased as the content of CNCs increased.
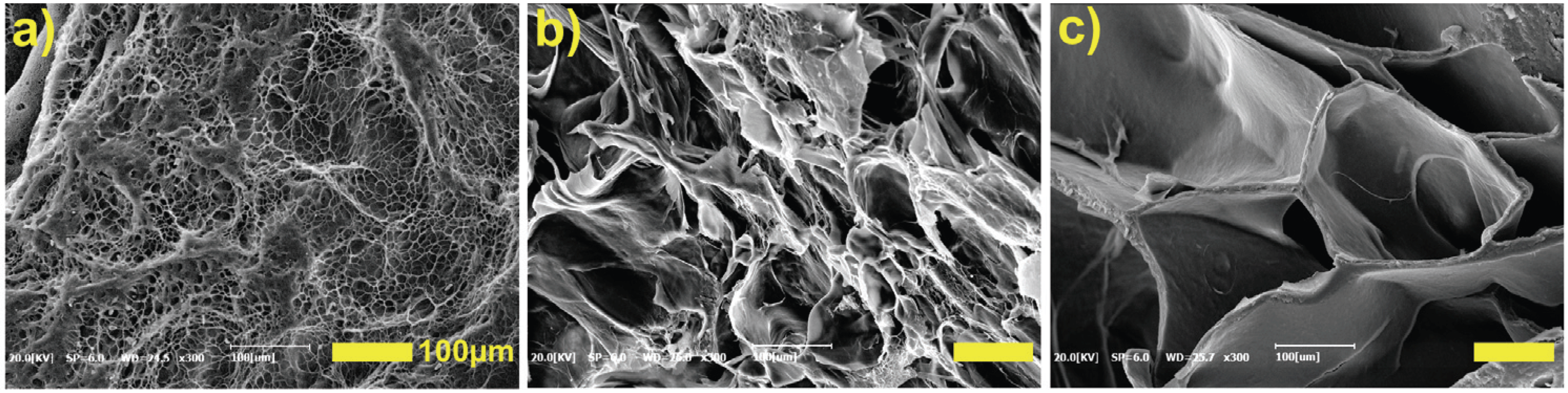
The hydrogels play a role as a release control through an expansion of polymer chains by water absorption, and it affects drug diffusion, especially release behavior. The release rate is determined by an ability of solvent molecules to move through the gel network, and the structure morphologically changes during the gel is swollen by diffusion through the polymer chains and strain relaxation between the chains (Mansur et al., 2008). Therefore, it is important to understand water content and swelling ratio properties for the following salicylic acid drug release behavior study. The water content and swelling ratio of the hydrogels were evaluated, and the properties change due to water absorption was observed. The results are presented in Fig. 4. As the CNCs content increased from 0, to 10 and 20 phr, the water content decreased from 91.2 to 90.0 and 89.4% and the swelling ratio from 306 to 290 and 272%. Noteworthy, both water content and swelling ratio tend to slightly decrease as the CNCS increase. As found in Fig. 2, the addition of CNCs is supposed to increase hydrophilic free-OH groups in the light of surface affinity perspective. Contrary to expectations, however, the water content decreased as the CNCs increased. The decrease of the swelling ratio can be affected by a spatial restrictioninside the hydrogels. In the case of a polymer mixed with a good solvent, as the chain entanglement is detangled and loosened, they can move freely and allows a large amount of solvent molecules to get in the spaces between the chains. Accordingly, the chains occupy morefree space, and when solid particles with high crystallinity are inserted between the polymer chains, their movement decrease, reducing their freedom. Especially, the similar characteristic of the surface between CNCs and PVA enhances its bonding, so the internal structure of the prepare hydrogels can be denser and more compact (Masruchin et al., 2015). For that reason, it was concluded that addition of CNCs inhibited PVA chain mobility, leading to a decrease in water content and swelling ratio. In terms of drug delivery, the higher the amount of water the hydrogels contain, the more drugs hydrogelscan store inside (Hoare and Kohane, 2008). Adding CNCs tends to speed up the initial water absorption because of many free-OH groups on the surface (Masruchin et al., 2015), but it has no positive effect on improving drug loading by volume increment of hydrogels.
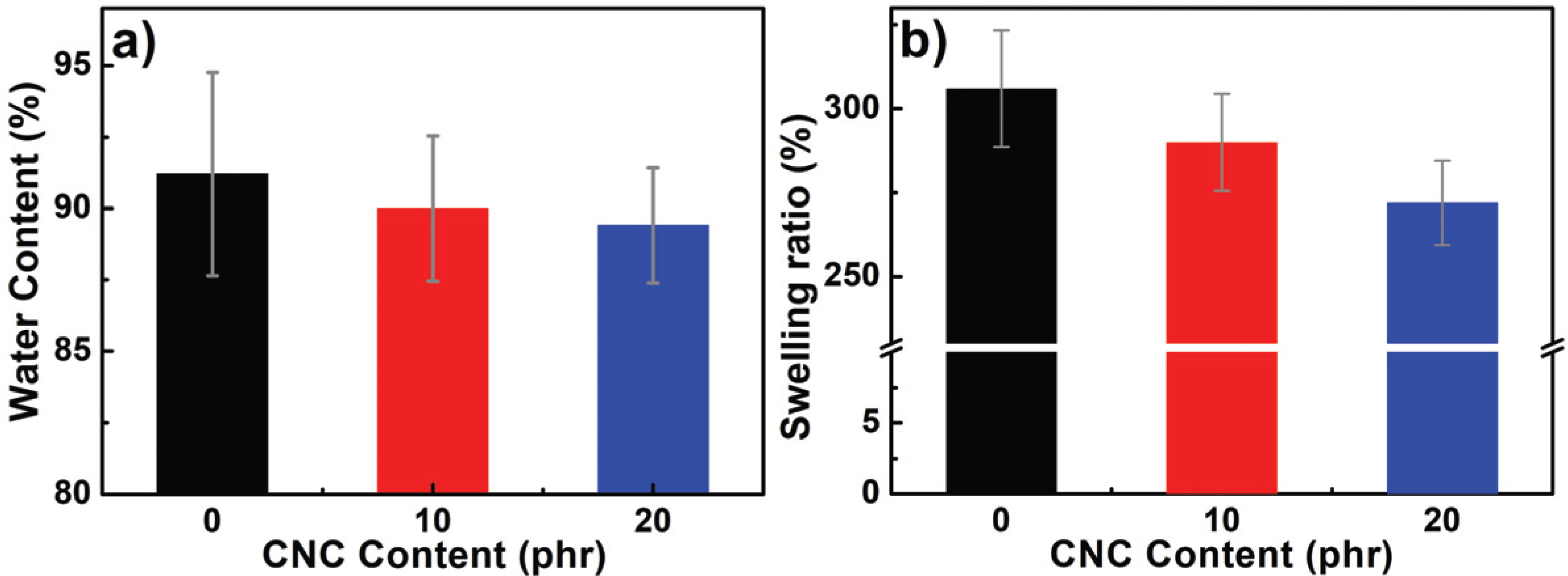
Storage modulus (G') and loss modulus (G") were studied by viscoelastic propertyanalysis, and the mechanical properties of CNCs-based PVA hydrogels were described in Fig. 5. It was founded that G' had a higher value that G" over the change of ω (angular frequency), and the prepared hydrogels have strong solid behavior (Yue et al., 2016). As it was explained in Section 3.1, it was caused by the formation of a 3D scaffold network (semi-IPN) cross-linked between acetal, ether, and hydrogen and polymer chains. Especially, all the viscoelasticity properties tend to increase with CNC content, which is caused by the CNCs with a large specific surface and a large amount of hydroxyl groups acting as a reinforcement by penetrating between PVA chains and strengthen physical and chemical binding and entanglement to restrict the molecule chains and distribute stress on the hydrogels and improve mechanical properties. Also, it was noted that a certain level of linear viscoelastic region (LVR) can be formed regardless of ω. If the forces applied inside the molecules are concentrated in a specific area without uniform dispersion, non-constant and irregular fluctuation of G' may occur due to complete or partial destruction of the intramolecular or intermolecular bonds at low ω (Mezger, T., 2016, Yue et al., 2016). Consequently, the stable formation of LVR throughout the ω region in hydrogels with CNCs implies that CNCs are evenly distributed between the PVA chains.
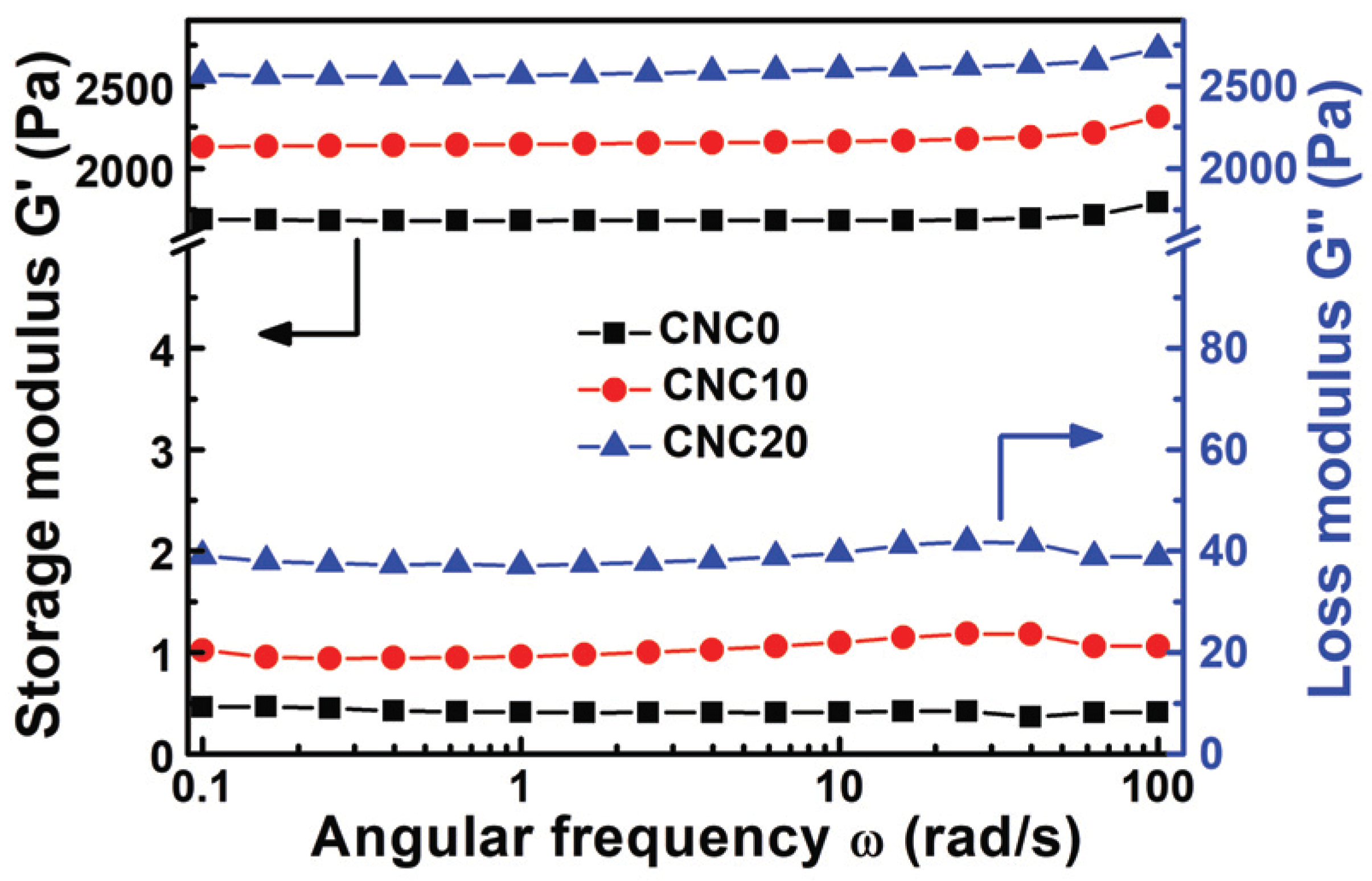
In this study, the drug loading performance was investigated using Langmuir isotherm and Freundlich isotherm as adsorption isotherm models. And the results are shown in Fig. 6a with salicylic acid loading amount by different concentration and the CNCs content. It was confirmed that moresalicylic acid was loaded on hydrogels with the CNCs than those without the CNCs, and the hydrogels with 10 phr added loaded a slightly more amount than those with 20 phr added. First of all, similar to the water content and swelling ratio characteristics mentioned above, it was founded that, despite the hydrogel without CNCs having more internal space, the hydrogel with CNCs loaded more drugs. Generally, the amount of adsorption increases on the interface as the surface area increases on the surface bonding mechanism (Zhou et al., 2015; Gao et al., 2017). From these results, the specific surface and pore volume were derived to calculate the surface are to pore volume ratio by the Baunauer-Emmett- Teller (BET) equation usingnitrogen adsorption/desorption method described in Section 2.3.1, and the results are presented in Fig. 7. Surface areasper unit volume (1 cm3) were 314, 677, and 61 m2 when respectively CNC0, CNC10, and CNC20 were added into the hydrogels. From these results, CNC10 was able to adsorb a large amount of the drug and the inner surface area got increased by addition of CNC even though it has less space inside as explained previously with water content and swelling ratio. On the contrary, comparing CNC0 with CNC20, CNC20 had a larger inner surface area than CNC0, but showed a less amount of adsorption. As shown in FT-IR results, the drug loading action point of hydrogel increased due to free-OH on CNCs surface with the addition of CNCs.
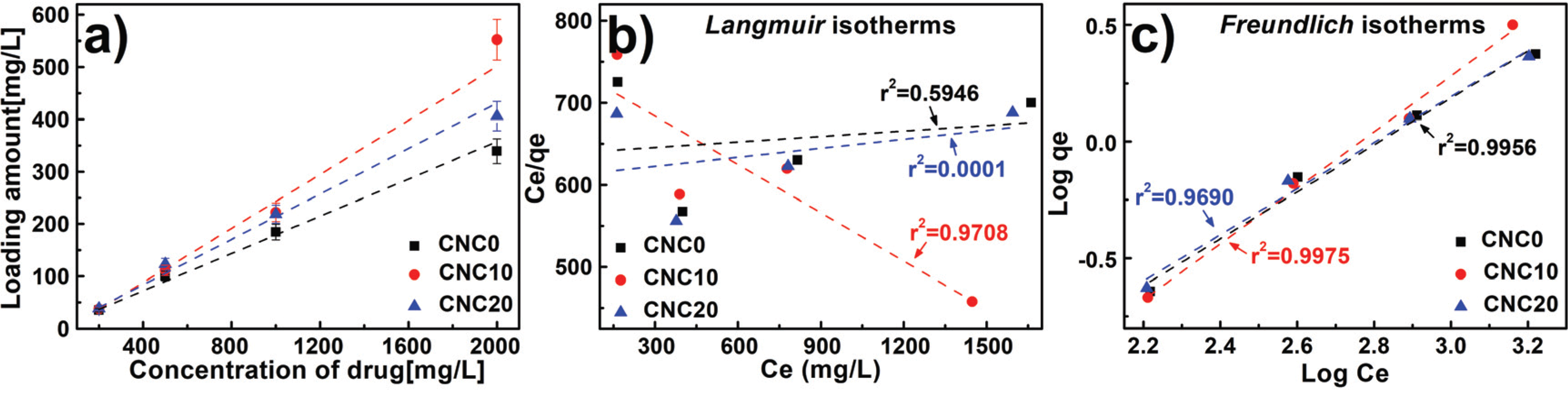
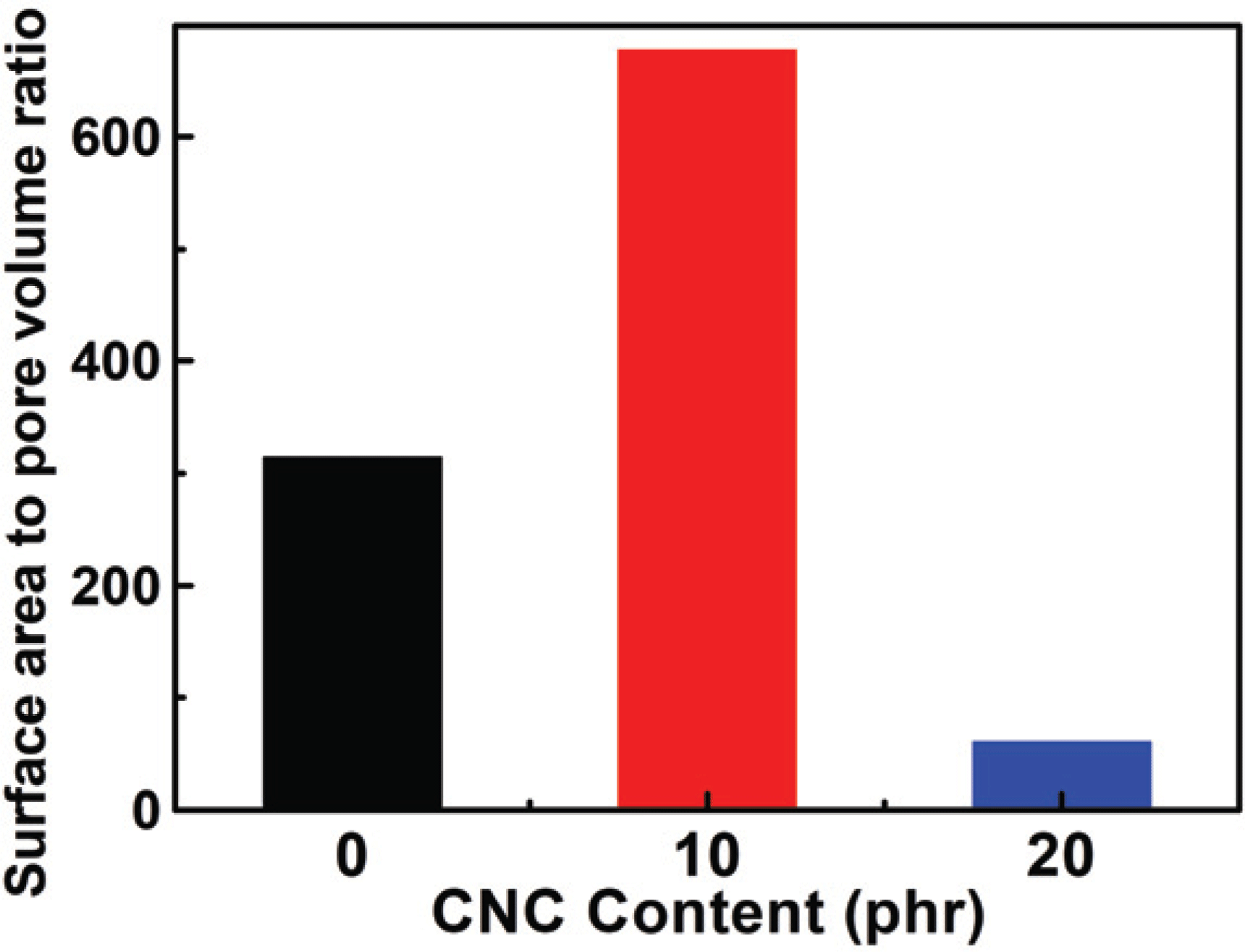
When comparing the drug loading performance of the hydrogels to which CNC10 and CNC20 were applied, it was confirmed that the loading efficiency of CNC20 was reduced compared to that of CNC10. It may be explained by a decrease of inner surface area and adsorption mechanism. To understand the mechanism of adsorption inside the hydrogels for drug loading, it was applied to Langmuir and Freundlich isotherm described in Section 2.3.5. The results are shown in Figs. 6b. and 6c. The correlation coefficients (r2: correlation coefficients) of CNC0, CNC10, and CNC20 calculated by Langmuir isotherm were 0.5946, 0.9708, and 0.0001. Also, it was calculated to be 0.9956, 0.9975, and 0.9690 by Freundlich isotherm, therefore, it was confirmed that Freundlich isotherm is more suitable for the salicylic acid loading mechanism of CNCs-based PVA hydrogels than Langmuir isotherm. Therefore, the drug ions, with the salicylic acid molecules on the inner interface of the hydrogels, were layered ina multi-molecule layer (Freundlich isotherm) instead of being bound as a mono molecular layer (Langmuir isotherm). It was confirmed that they were stacked in a multi-molecule layer due to electrical action that is on various pore interfaces inside and on interface between a drug ion and another, and differences in electrical strength such as radicals, van der Waals forces, etc. (Cho et al., 2013; Tilak et al., 2016; Li and Mooney, 2016). It shows that it needs enough space to load by forming a multi-molecular layer on the surface as a drug is loaded in a chemical and physical way on CNCs-based PVA hydrogels. To evaluate adsorption capacity, it can be presented in Table 3 with Freundlich constant KF from the equation (6) and experiment constant nfrom Freundlich isotherm. At KF and n which represent adsorption performance, CNC10 showed higher values than CNC20, confirming that CNC10 was a more favorable condition for drug loading. In conclusion, to increase drug loading amount by improving the mechanical strength on CNCs-based PVA hydrogels, a content of CNCs should be optimized and application of CNC10 is more beneficial.
n | KF | |
---|---|---|
CNC0 | 0.9530 | 0.0244 |
CNC10 | 1.2241 | 0.0497 |
CNC20 | 1.0653 | 0.0287 |
A drug release behavior applied to the hydrogels has a significance in the light of an effective use of the drug. The drug release properties of CNCs-based PVA hydrogels were characterized and the results are presented in Fig. 8a. As expected by performance analysis of adsorption, the hydrogels applied with CNC10 having a high loading capacity reached to equilibrium at high temperatures with large release amounts. The results were described in Fig. 8b. and 8c; all release data was applied to the pseudo 1st order model and the pseudo 2nd order model to evaluate release speed. By comparing r2: correlation coefficient of a trend line, it was confirmed that the pseudo 2nd order model was more suitable than the pseudo 1st order model. The reaction speed constant k2 and h, which means the initial release speed, were calculated for comparative analysis of drug release rate through the the pseudo 2nd order model and the results were shown in Table 4. When CNCs were added, it showed that ‘h’ decreased, which represents the initial released speed of the prepared hydrogels. This demonstrates delayed release of drug by drug adsorption function of CNCs. When it was not added, most drugs were simply diffused by osmotic because they were mainly diluted instead of being combined on the inner surface of the hydrogels. On the other hand, it suggests that the CNCs-added hydrogels had a delayed release speed of the drug because of many acting points on CNCs surface and physical and chemical bonds among drug ions (Li et al., 2016). Accordingly, it was concluded that CNCs-added hydrogels had a positive effect on delayed release of the drug.
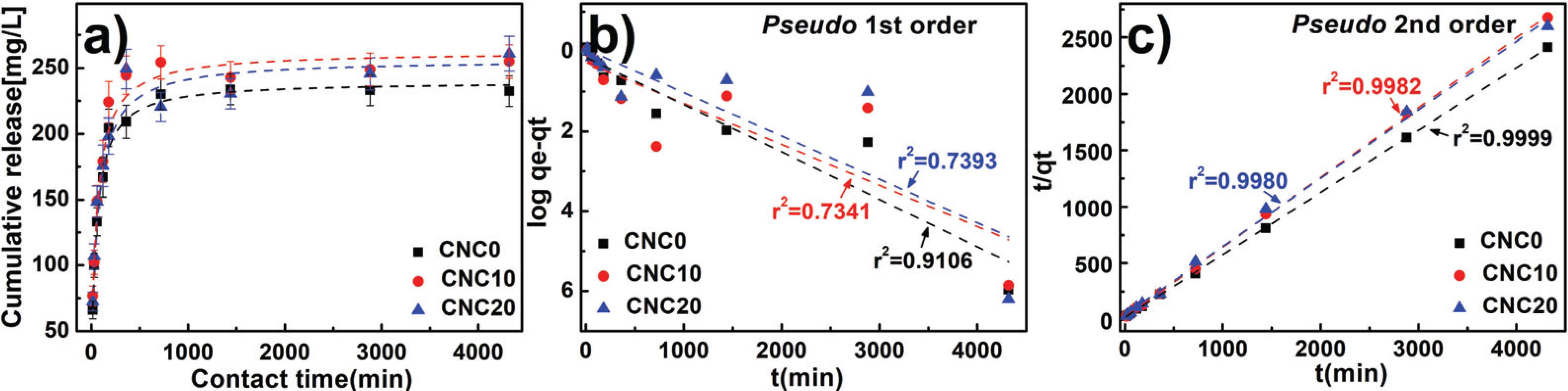
Pseudo 1st order model | Pseudo 2nd order model | ||
---|---|---|---|
CNC0 | k1 = 0.0028 | k2 = 0.0148 | h = 0.0483 |
CNC10 | k1 = 0.0023 | k2 = 0.0106 | h = 0.0263 |
CNC20 | k1 = 0.0025 | k2 = 0.0089 | h = 0.0242 |
4. CONCLUSION
CNCs-based PVA hydrogels were prepared, and its physical, chemical and mechanical properties were investigated. Also, salicylic acid as a drug model was applied to investigate the adsorption and release properties of hydrophobic drugs, and the interaction between the drug and the hydrogels was evaluated using various dynamic models. The FT-IR results showed that inner PVA hydrogels with GA as a cross-linking agent formed a 3D scaffold cross-linked network (semi-IPN), and it allowed a strong and dense 3D structure by adding CNCs. These characteristics of CNCs involved that water content and swelling ratio have decreased, andviscoelasticity has increased as the mechanical properties. In addition, from the analysis of drug loading behavior, Langmuir isotherm and Freundlich isotherm were applied, and it was found that Freundlich isotherm is more suitable than Langmuir isotherm. From this study, it has been loaded ina multi-molecular layer multiplied by physical and chemical bonds inside the hydrogels, not a mono-molecular layer of the drug. The addition of CNCs increased hydroxyl groups that did not involve with the formation of the 3D network structure,increasing drug loading amounts. Besides, CNC10 having a larger inner specific surface than CNC20 showed ahigher loading capacity. Also, the drug release properties analysis found the effect of delayed release of a drug and a high potential of crystalline nanocellulose CNCs as a core material of the delayed release drug delivery system.